- Search Menu
- Volume 11, Issue 4, April 2024 (In Progress)
- Volume 11, Issue 3, March 2024
- Advance articles
- Editor's Choice
- Insight Articles
- Novel ID Cases and Teaching Cases
- Supplement Archive
- Cover Archive
- IDSA Journals
- Clinical Infectious Diseases
- The Journal of Infectious Diseases
- Author Guidelines
- Open Access
- Why Publish
- About Open Forum Infectious Diseases
- About the Infectious Diseases Society of America
- About the HIV Medicine Association
- IDSA COI Policy
- Editorial Board
- Advertising and Corporate Services
- Journals Career Network
- Self-Archiving Policy
- For Reviewers
- For Press Offices
- Journals on Oxford Academic
- Books on Oxford Academic
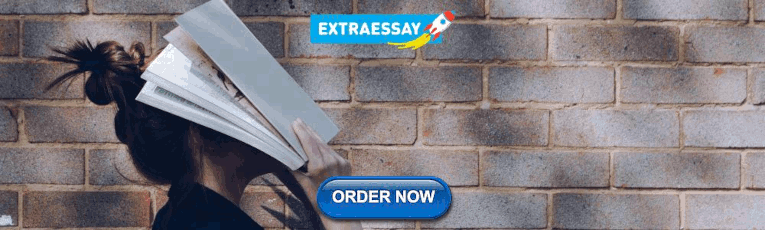
Article Contents
The number of influenza risk factors informs an adult's increased potential of severe influenza outcomes: a multi-season cohort study from 2015 to 2020.

- Article contents
- Figures & tables
- Supplementary Data
Ian McGovern, Katherine Cappell, Alina N Bogdanov, Mendel D M Haag, The Number of Influenza Risk Factors Informs an Adult's Increased Potential of Severe Influenza Outcomes: A Multi-Season Cohort Study from 2015 to 2020, Open Forum Infectious Diseases , 2024;, ofae203, https://doi.org/10.1093/ofid/ofae203
- Permissions Icon Permissions
While studies have evaluated individual factors influencing the risk of severe influenza outcomes, there is limited evidence on the additive impact of having multiple influenza risk factors and how this varies by age.
Patients ≥18 years of age in the US were evaluated retrospectively in five seasonal cohorts during the 2015–2020 influenza seasons. Patient-level electronic medical records linked to pharmacy and medical claims were used to ascertain covariates and outcomes. Multivariable logistic regression models were fitted for the overall population and age subgroups to evaluate the association of demographic and clinical characteristics with odds of influenza-related medical encounters (IRME) (ICD-10 codes J09*–J11*). The logistic regression models included sex, race/ethnicity, geographic region, baseline healthcare resource use, vaccination status, specific high-risk comorbidities, number of influenza-risk factors, BMI, and smoking status. Odds ratios (OR) from each of the five individual seasons were summarized using fixed-effects meta-analysis.
Season cohort sizes ranged from 887,260 to 3,628,168 individuals. Of all patient characteristics evaluated, the cumulative number of CDC-defined high-risk influenza conditions that an individual had was most predictive of increased probability of having an IRME overall and across age groups, with adults of any age with ORs for influenza hospitalization ranging from 1.8 (95%CI: 1.7 to 2.0) for 1 risk factor up to 6.4 (95%CI: 5.8 to 7.0) for individuals with ≥4 risk-factors.
These results show that a simple measure like the number of influenza risk factors can be highly informative of an adult’s potential for severe influenza outcomes.
Supplementary data
Email alerts, citing articles via, looking for your next opportunity, affiliations.
- Online ISSN 2328-8957
- Copyright © 2024 Infectious Diseases Society of America
- About Oxford Academic
- Publish journals with us
- University press partners
- What we publish
- New features
- Open access
- Institutional account management
- Rights and permissions
- Get help with access
- Accessibility
- Advertising
- Media enquiries
- Oxford University Press
- Oxford Languages
- University of Oxford
Oxford University Press is a department of the University of Oxford. It furthers the University's objective of excellence in research, scholarship, and education by publishing worldwide
- Copyright © 2024 Oxford University Press
- Cookie settings
- Cookie policy
- Privacy policy
- Legal notice
This Feature Is Available To Subscribers Only
Sign In or Create an Account
This PDF is available to Subscribers Only
For full access to this pdf, sign in to an existing account, or purchase an annual subscription.
ORIGINAL RESEARCH article
Trivalent mrna vaccine-candidate against seasonal flu with cross-specific humoral immune response.
- 1 Federal State Budget Institution “National Research Centre for Epidemiology and Microbiology Named after Honorary Academician N. F. Gamaleya” of the Ministry of Health of the Russian Federation, Moscow, Russia
- 2 Department of Virology, Lomonosov Moscow State University, Moscow, Russia
- 3 Department of Medical Genetics, I. M. Sechenov First Moscow State Medical University, Moscow, Russia
- 4 Shemyakin-Ovchinnikov Institute of Bioorganic Chemistry of the Russian Academy of Sciences, Moscow, Russia
- 5 Belozersky Institute of Physico-Chemical Biology, Lomonosov Moscow State University, Moscow, Russia
- 6 Faculty of Bioengineering and Bioinformatics, Lomonosov Moscow State University, Moscow, Russia
- 7 Translational Medicine Research Center, Sirius University of Science and Technology, Sochi, Russia
- 8 Infectiology Department, I. M. Sechenov First Moscow State Medical University, Moscow, Russia
Seasonal influenza remains a serious global health problem, leading to high mortality rates among the elderly and individuals with comorbidities. Vaccination is generally accepted as the most effective strategy for influenza prevention. While current influenza vaccines are effective, they still have limitations, including narrow specificity for certain serological variants, which may result in a mismatch between vaccine antigens and circulating strains. Additionally, the rapid variability of the virus poses challenges in providing extended protection beyond a single season. Therefore, mRNA technology is particularly promising for influenza prevention, as it enables the rapid development of multivalent vaccines and allows for quick updates of their antigenic composition. mRNA vaccines have already proven successful in preventing COVID-19 by eliciting rapid cellular and humoral immune responses. In this study, we present the development of a trivalent mRNA vaccine candidate, evaluate its immunogenicity using the hemagglutination inhibition assay, ELISA, and assess its efficacy in animals. We demonstrate the higher immunogenicity of the mRNA vaccine candidate compared to the inactivated split influenza vaccine and its enhanced ability to generate a cross-specific humoral immune response. These findings highlight the potential mRNA technology in overcoming current limitations of influenza vaccines and hold promise for ensuring greater efficacy in preventing seasonal influenza outbreaks.
1 Introduction
Seasonal influenza is a highly contagious respiratory disease caused by influenza A and B viruses that circulate around the world. Globally, influenza causes ~1 billion cases of illness, 3 to 5 million cases of severe illness, and up to 500,000 deaths annually ( 1 ). Pregnant women, children aged 6 months to 5 years, elderly people (aged more than 65 years), individuals with chronic disease, and healthcare workers are at increased risk of severe illness and serious complications from influenza virus infection ( 2 , 3 ). A comprehensive study employing regression models revealed that the mortality rate associated with influenza between 1990 and 2017 was most pronounced among individuals over 70 years old, with a rate of 16.4 deaths per 100,000 (95% CI 11.6-21.9) ( 4 ). Vaccination remains the primary strategy for reducing the incidence of influenza.
Various types of flu vaccines are available, including live attenuated, inactivated (whole virion, split, subunit), and recombinant vaccines ( 5 ). The effectiveness of these vaccines (i.e. their ability to provide protection against influenza) may vary from season to season. At least two factors determine the likelihood of vaccine efficacy: (i) characteristics of the individual being vaccinated, such as age and health status, and (ii) the degree of matching between the vaccine composition and the influenza strains currently circulating in the human population ( 6 ). Currently, most influenza vaccines are either quadrivalent (containing antigens of the H1N1 and H3N2 strains of influenza A combined with two lineages of influenza B, including the Victoria and Yamagata variants), or trivalent (containing the influenza A antigens of the H1N1 and H3N2 subtypes and one of the two influenza B subtypes) ( 7 ). According to the CDC, in seasons when vaccine antigens matched circulating influenza viruses, vaccination reduced the risk of doctor visits related to influenza by 40% to 60% ( 8 ). A 2021 study reported that among adults, vaccination was associated with a 26% lower risk of intensive care unit (ICU) admission and a 31% lower risk of death from influenza compared with those who were not vaccinated ( 9 ). From 2010 to 2012, vaccination led to a 74% reduction in the risk of influenza-related ICU admissions in children ( 10 ), and according to a 2017 study, vaccination reduced the risk of influenza-related hospitalizations in older adults by an average of 40% between 2009 and 2016 ( 11 ). Thus, vaccine prevention of influenza is both effective and justified.
In contrast, in instances where there were errors in selecting the appropriate antigenic composition, the efficacy of the vaccine was significantly compromised. A notable example was the 2017/18 vaccine, which exhibited low efficacy (~25%) in the UK due to a mismatch with the predominant influenza A strain ( 11 ). A similar decrease in effectiveness (to as low as 13%) relative to the H3N2 component of the vaccine was observed during the 2014-2015 season ( 12 ). Throughout the history of influenza vaccination, there have been numerous occurrences of such mismatches, resulted in elevated rates of severe illness and mortality from influenza in certain seasons. To mitigate the impact of seasonal and pandemic influenza on public health, there is a need for vaccines that would offer broader and more reliable protection ( 13 ).
Different approaches and platforms have been employed in the development of new influenza vaccines, including virus-like particles, DNA/mRNA vaccines, baculovirus expression system, viral vectors, et al. Of particular interest are mRNA-based vaccines, which have demonstrated their efficacy and safety during the COVID-19 pandemic ( 14 , 15 ). To date, a high immunogenicity of candidate mRNA influenza vaccines in animals and humans have been reported in a few studies ( 16 – 19 ). In particular, immunization of mice with an mRNA candidate vaccine containing mRNAs encoding twenty hemagglutinins (HAs) of various influenza virus strains led to the formation of a prolonged humoral response to all twenty HAs ( 16 ). Notably, the multivalent vaccine showed robust protection in animal models (mice, ferrets) when challenged with H1N1 influenza strains that varied in their similarity to the vaccine strain. The authors reported no mortality among vaccinated animals and observed a reduced disease severity (clinical scores) and a significantly lower weight loss compared to the control group ( 16 ).
A team led by G. Ciaramella has conducted extended preclinical and phase I clinical trials to evaluate the immunogenicity of modified mRNA encoding HA proteins from avian influenza viruses (H7 and H10) formulated in lipid nanoparticles (LNP) ( 19 ). In mice, these vaccines demonstrated a 2-5-fold increase in hemagglutination inhibition assay (HAI) titers on day 21 after immunization, which remained at a consistent level throughout the year. The protective effect of the H7-mRNA was observed even with a minimal vaccine dose (0.4 μg per mouse), although it strongly depended on the period between immunization and infection (shorter intervals led to more rapid weight loss and their death from infection in vaccinated animals). Immunization of non-human primates with a single dose of 400 μg of H10- or H7-mRNA generated an immune response with HAI titers in serum ranging from 1:100 to 1:1 000; two weeks after repeated immunization, HAI titers reached 1:1 000 000. In two randomized, double-blind, placebo-controlled phase 1 studies involving healthy volunteers (n=201 for H10-mRNA and n=165 for H7-mRNA), the vaccines demonstrated favorable safety and reactogenicity profiles, as well as a robust humoral immune response ( 20 ). Following double intramuscular immunization with 100 µg of H10-mRNA, all volunteers exhibited serum HAI titers exceeding 1:40, and 87% of participants showed microneutralization reaction titers of ≥1:20. For H7-mRNA, intramuscular administration of 10, 25, and 50 µg doses led to HAI titers exceeding ≥1:40 in ~36%, 96%, and 90% of participants, respectively. At the same time, no significant HA-specific cellular immune response was observed in the IFN-γ ELISPOT assay ( 20 ).
Despite the growing body of research on the immunogenicity of mRNA vaccines against influenza, there is still a limited understanding of their potential to elicit a broad immune response against influenza strains with varying degrees of homology. In this study, we addressed this issue by presenting our own experience in the development of a trivalent mRNA flu vaccine and exploring its immunogenicity and protective efficacy in a mouse model. Through a two-dose immunization of mice, we observed not only a robust humoral immune response, but also cross-reactivity of this response against heterologous strains of the influenza virus.
2 Materials and methods
2.1 mrna production.
The pJAZZ-OK-based linear bacterial plasmids (Lucigen) with coding regions of every HAs were used as templates for mRNAs production. DNA cloning procedures were performed as described earlier ( 21 ). The identity of the coding sequences was confirmed by Sanger sequencing. The pDNA for IVT were isolated from the E.coli BigEasy™-TSA™ Electrocompetent Cells (Lucigen) using the Plasmid Maxi Kit (QIAGEN). The pDNA was digested using BsmBI-v2 restriction endonuclease (NEB), followed by purification of the product by phenol-chloroform extraction and ethanol precipitation. IVT was performed as described earlier ( 21 ). Briefly, 100-μl reaction volume contained 3 μg of DNA template, 3 μl T7 RNA polymerase (Biolabmix) and T7 10X Buffer (TriLink), 4 mM trinucleotide cap 1 analog (3′-OMe-m7G)-5′-ppp-5′-(2′-OMeA)pG (Biolabmix), 5 mM m1ΨTP (Biolabmix) replacing UTP, and 5 mM GTP, ATP and CTP. After 2 h incubation at 37°C, 6 μl DNase I (Thermo Fisher Scientifiс) was added for additional 15 min, followed by mRNA precipitation with 2M LiCl (incubation for 1 h in ice and centrifugation for 30 min at 14,000 g, 4°C) and carefully washed with 80% ethanol. RNA integrity was assessed by electrophoresis in 8% denaturing PAGE.
2.2 mRNA-LNP assembly
LNP assembly was performed as described earlier ( 21 ) with some modifications. In brief, all lipid components were dissolved in ethanol at molar ratios 46.3:9:42.7:1.6 (ionizable lipid:DSPC:cholesterol:PEG-lipid). Acuitas ionizable lipid (ALC-0315) and PEG-lipid (ALC-0159) were purchased in Cayman Chemicals. The lipid mixture was combined with an acidification buffer of 10 mM sodium citrate (pH 3.0) containing mRNA (0.2 mg/mL) at a volume ratio of 3:1 (aqueous: ethanol) using the NanoAssemblr Ignite device (Precision NanoSystems). The ratio of ionizable nitrogen atoms in the ionizable lipid to the number of phosphate groups in the mRNA (N:P ratio) was set to 6 for each formulation. Formulations were dialyzed against PBS (pH 7.2) in Slide-A-Lyzer dialysis cassettes (Thermo Fisher Scientific) for at least 24 h. Formulations were passed through a 0.22-μm filter and stored at 4°C (PBS) until use. The diameter and size distribution, zeta potential of the mRNA-LNP were measured using a Zetasizer Nano ZS instrument (Malvern Panalytical) according to user manual.
The mRNA encapsulation efficiency and concentration were determined by SYBR Green dye (SYBR Green I, Lumiprobe) followed by fluorescence measurement. Briefly, mRNA-LNP samples were diluted with TE buffer (pH 8.0) in the absence or presence of 2% Triton-X-100 in a black 96-well plate. Standard mRNA (4 ng/μL) was serially diluted with TE buffer in the absence or presence of 2% Triton-X-100 to generate standard curves. Then the plate was incubated 10 min at room temperature on a rotating shaker (260 rpm) followed by addition of SYBR Green dye (100 times diluted in TE buffer) to each well to bind RNA. Fluorescence was measured at 454 nm excitation and 524 nm emission using Varioscan LUX (Thermo Fisher Scientifiс). The concentrations of mRNA after LNP disruption by Triton-X-100 (C total mRNA ) and before LNP disruption (C outside mRNA ) were determined using the corresponding standard curves. The concentration of mRNA loaded into the LNP was determined as the difference between the two concentrations multiplied by the dilution factor of the original sample. Encapsulation efficiency was calculated by the formula:
2.3 Cell culture
HEK293 cells (ATCC CRL-1573) were cultured in Dulbecco’s modified Eagle’s medium (DMEM, Paneco) supplemented with 2 mM L-Glutamine (Gibco), 10% fetal bovine serum (HyClone), 50 U/mL penicillin and 50 μg/mL streptomycin (both from Paneco). Transfection of HEK293 cells with H1N1 HA-encoding mRNA was performed as described previously ( 21 ) with minor modification. Briefly, HEK293 cells were plated on a 12-well plate in a density of 2×10 5 cells per well and maintained at 37°C in 5% CO 2 . The next day the medium was replaced with a fresh DMEM without antibiotics and cells were transfected by HA-mRNA using Lipofectamine 3000 reagent (Invitrogen) and Opti-Mem I Reduced Serum Medium (Gibco) in accordance with the manufacturer’s instructions. 24 h after transfection, cells were analyzed by immunocytochemical staining.
2.4 Immunocytochemistry (ICC)
For the analysis of the HA expression in the transfected HEK293 cells, they were fixed in 4% PFA (paraformaldehyde) for 30 min at 40°C, washed with PBS and permeabilized in 0.1% Triton X-100. The cells were then incubated with primary goat Anti-Influenza A Antibody (Chemicon ® , Sigma-Aldrich, #AB1074) in PBS with 0.1%/0.02% BSA/Triton X-100 at 40°C overnight. The next day, cells were incubated with Donkey Anti-Goat IgG H&L (Alexa Fluor ® 488) (ab150133; Abcam) secondary antibodies for 1 h at room temperature. Nuclei were stained with 4,6-diamidino-2-phenylindole (DAPI) (300 nM). Images were acquired on ZOE Fluorescent Cell Imager (Bio-Rad).
2.5 Viruses
Influenza virus propagation in embryonated chicken eggs or MDCK cells was performed according to conventional technique as described earlier ( 22 , 23 ). Briefly, specific pathogen-free (SPF) fertilized 9-10 days old chicken eggs were purchased from Nursery “Podmoklovo” (Russia). Presence of the embryo was monitored using an egg candler. Virus inoculation is carried out by injection of virus stock into the allantoic cavity using a needle. After 2 days of incubation at 34°C, the eggs are cooled for at least 4 h at 4°C. The eggshell above the air sac and the chorioallantoic membrane are then carefully opened, and the allantoic fluid containing the virus is harvested. The fluid is cleared from debris by centrifugation, aliquoted and transferred to -80°C for long-term storage. Virus titer was determined by endpoint dilution assay on MDCK cells as previously described ( 23 ).
2.6 Animal studies
Females BALB/c mice of 4-5 weeks old were used for the immunogenicity study of HA-mRNA-LNPs and for the viral challenge experiments. Animals were purchased from Stolbovaya breeding and nursery laboratory (Research Center for Biomedical Technologies of FMBA; Russia). All animal experiments were performed in accordance with the Directive 2010/63/EU, FELASA recommendations ( 24 ), and the Inter-State Standard of “GLP” ( 25 ) approved by the Institutional Animal Care and Use Committee (IACUC) of the Federal Research Centre of Epidemiology and Microbiology named after Honorary Academician N.F. Gamaleya and were performed under Protocol #41 from 6 April 2023. All persons using or caring for animals in research underwent training annually as required by the Biomedical Ethics Committee.
2.7 Immunizations
For mouse intramuscular immunization 100 μl of vaccine was injected in either the left or right hindlimb muscles. Mice received two doses of HA-mRNA-LNPs (altogether or apart) with a 14- or 21-days interval, while the placebo group received PBS. Mice from the positive control group were injected by equal volume (100 μl) of 1/10 human dose of split inactivated influenza vaccine.
2.8 Hemagglutinin inhibition (HAI) assay
Immunogenicity in animal experiments was estimated by hemagglutinin inhibition assay, according to the World Health Organization (WHO)-based HAI protocol ( 26 ). Shortly, mouse sera were treated by a receptor destroying enzyme (RDE produced from Vibrio cholerae was purchased from Denka Seiken Co., Ltd., Tokyo, Japan), then twofold dilutions of treated sera to be tested are made in 96 well plates. The viral antigen was added, and the plate was incubated for 30 minutes at room temperature. Human red blood cells (RBC) type O are then added and the plate incubated for a further 60 minutes at room temperature. If there were antibodies in the serum sample that cross-reacted with the virus, the antibodies would bind to the virus and prevent the virus from hemagglutinating the RBC. After incubation, the HAI titer was red as the highest dilution of serum that inhibited hemagglutination. Antigens of influenza virus for HAI test (A/Darwin/9/2021, A/Victoria/2570/2019, A/Wisconsin/588/2019, B/Austria/1359417/2021, B/Phuket/3073/2013) were purchased in LLC “Company for the production of diagnostic drugs” (St-Petersburg, Russia) or propagated in embryonated chicken eggs (A/California/07/2019 pdm09, B/Washington/02/2019) or propagated in MDCK cells (A/Guangdong-Maonan/SWL1536/2019, A/Moscow/52/2022).
2.9 HA domain ELISAs
ELISA plates (96-well; Servicebio) were coated with 100 μL of recombinant proteins in PBS at 1 μg/mL and incubated overnight at 4°C. The day of the experiment, plates were blocked with 150 μL of S002X buffer (Xema, Moscow, Russia) and incubated 2 h at room temperature. Five-fold serial dilutions of samples in ELISA buffer S011 (Xema, Moscow, Russia) were added to the plates and allowed to incubate for 1 h at 37°C (initial sample dilution 1:10). Plates were then incubated with peroxidase-conjugated goat anti-mouse IgG (L20/01; HyTest; 1:25000) for 1 h at 37°C. After final wash chromogen substrate solution R055 (Xema, Moscow, Russia) was added to each well, and the reaction was then stopped with the addition of 100 mM HCl solution. Absorbance was read at 450 nm using plate reader (Multiscan FC, Thermo Scientific). Plates were washed 3 times after first incubation and 6 times after second incubation with wash buffer S008 (Xema, Moscow, Russia). A modified trimeric HA stem domain (influenza H1N1 A/Brisbane/59/2007) was a gift from Dr. Dmitry V. Shcheblyakov from the laboratory of immunobiotechnology of the National Research Centre for Epidemiology and Microbiology Named after Honorary Academician N. F. Gamaleya previously described at ( 27 ). The HA head domain (HA1 subunit of influenza A H1N1 A/California/04/2009) were purchased (Cat. 11055-V08H4 Sino Biological).
2.10 Viral challenge
The lethal infection caused by influenza virus was performed on 4-5-week-old female BALB/c mice. Mouse adapted influenza virus H1N1 A/Victoria/2570/2019 was obtained from the laboratory of molecular biotechnology of the Federal Research Centre of Epidemiology and Microbiology named after Honorary Academician N.F. Gamaleya. Mice were infected intranasally with 50 µL of virus suspension under Zoletil-Xylazine anesthesia. Animals were monitored for clinical symptoms (weight loss, survival) every day through 10 days after the challenge. Assessment of clinical symptoms was carried out in accordance with the scale: score of 0 (no symptoms), score of 1 (mild symptoms), score of 2 (moderate symptoms), score of 3 (severe symptoms = humane endpoint). Time of death was defined as the time at which a mouse was found dead or was euthanized via carbon dioxide asphyxiation followed by cervical dislocation at the endpoint.
2.11 Quantification of virus in infected lungs from mice
Lungs were harvested from mice 3 days post-infection. Following harvest, lungs were weighed, and then homogenized in sterile DMEM with gentamycin to generate a 20% lung-in-medium solution. Total RNA was extracted from lung homogenates using the ExtractRNA Reagent (Eurogen, Moscow, Russia) following the manufacturer’s instructions. Amplification and quantification of influenza A virus RNA were carried out by using a one-step RT-qPCR technique. To perform one-step RT-qPCR was used the reaction mixture containing (for one reaction) 5 pmol of each primer, 3 pmol of probe, 12.5 μl of 2xBioMaster RT-PCR-RT (Biolabmix, Moscow, Russia) and 10 μl of RNA (0.5 μg). The total volume of the one reaction mixture was 25 μl. The primers and probes were designed to target the gene coding M (matrix protein) of influenza A virus, the oligonucleotides were as follows: forward primer – 5’- ATG GAG TGG CTA AAG ACA AGA C -3’, reverse primer - 5’- GCA TTT TGG ACA AAG CGT CTA -3’, probe 5’-FAM - TCC TCG CTC ACT GGG CAC GGT -BHQ1-3’. Amplification was performed using a Real-time CFX96 Touch instrument (Bio-Rad, USA). The conditions of the one-step RT-qPCR reaction were as follows: 50°C for 15 min, 95°C for 5 min, followed by 45 cycles of 95°C for 10 s and 55°C for 1 min. The number of copies of viral RNA was calculated using a standard curve generated by amplification of a plasmid cloned DNA template containing the amplified fragment.
2.12 Statistical analysis
Data were analyzed using GraphPad Prism software version 9.5.0. Data of immunogenicity were analyzed using a Kruskal-Wallys test with Dunn’s multiple comparison test for inter-group analysis and Friedman test with Dunn’s comparison test for intra-group analysis of HAI titers through the time. Survival data were compared using the Mantel-Cox long-rank test with Dunn’s multiple comparison test and weight loss was compared using Tukey’s multiple comparison test. The viral load data were compared using Mann-Whitney test.
3.1 Preparation and characterization of mRNA vaccine compositions
To develop the vaccine, HAs of three influenza viruses were chosen: A/Wisconsin/588/2019 (H1), A/Darwin/6/2021 (H3), B/Austria/1359417/2021 (IBV, Victoria lineage). These strains of influenza virus were included in the WHO recommendations for the 2022-2023 seasonal influenza vaccine in the northern hemisphere ( 28 ). Codon optimized DNA sequences of HA genes were synthesized and cloned into the pJAZZ-OK linear bacterial plasmid, as described previously ( 21 ). In vitro synthesized mRNAs (schematically shown in Figure 1 ) included the cap-1 structure at the 5′ end; a 100-nt long poly(A)-tail at the 3′ end; the 5′ and 3′ untranslated regions (UTRs) from the human hemoglobin alpha subunit (HBA1) mRNA; and codon optimized coding sequences (CDS).
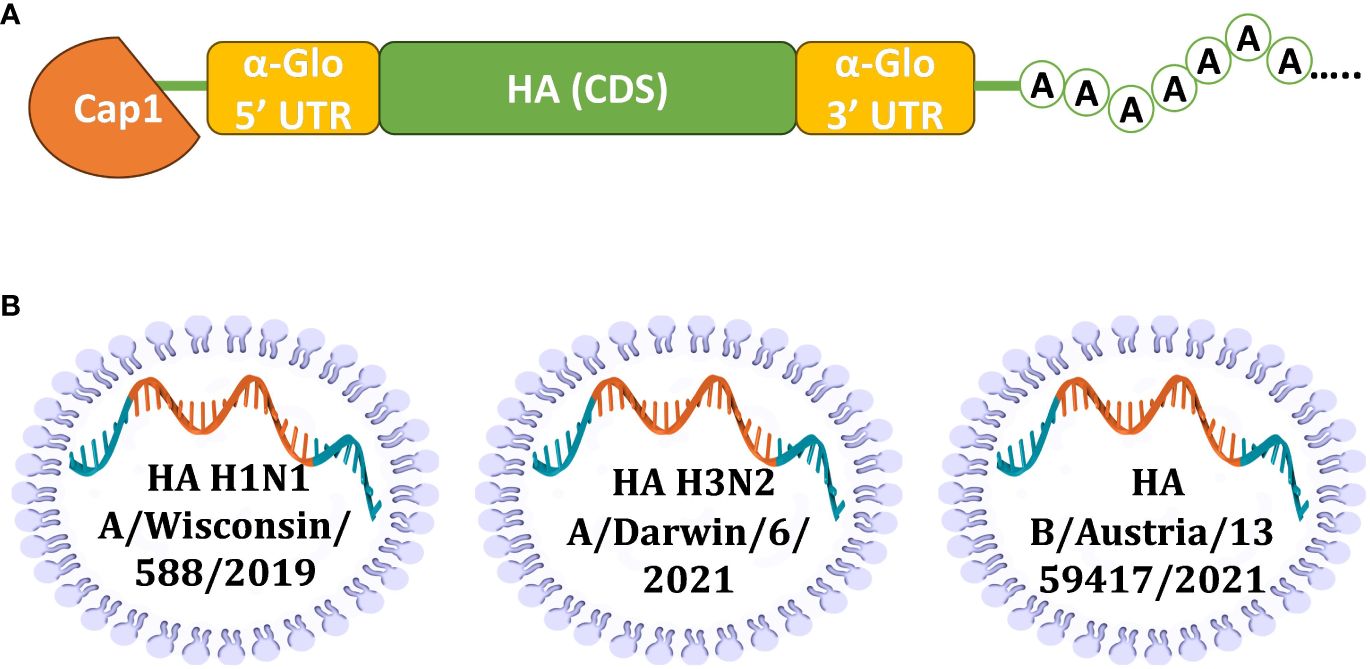
Figure 1 Design of mRNA-LNP for effective production of HA antigens in mammalian cells. (A) Scheme of mRNA with key parts, including 5’ and 3’ UTRs, influenza virus (IV) HA CDS, and poly(A)-tail; (B) Schematic visualization of separately formulated mRNAs encoding influenza HAs from three seasonal (2022-2023) vaccine strains.
N1-methylpseudouridines (m1Ψ) were co-transcriptionally incorporated into the mRNA instead of 100% uridines (U). mRNA-LNP formulations were prepared using the microfluidic NanoAssemblr Ignite mixer. The encapsulation efficiency was 89% (SD 1.2%) with a typical average particle size in the range of 68-71 nm with 0.105-0.148 polydispersity index ( Supplementary Table S1 ).
A proper expression of the in vitro synthesized mRNAs was confirmed by transfection of cultured human embryonic kidney cells (HEK293) followed by their immunocytochemical staining for the H1N1 HA product. The presence of the HA protein (H1N1 A/Wisconsin/588/2019) was detected both inside the cells (intracellular staining, Figures 2A, C ) and in the membrane-associated form (surface cell staining, Figures 2B, D ).
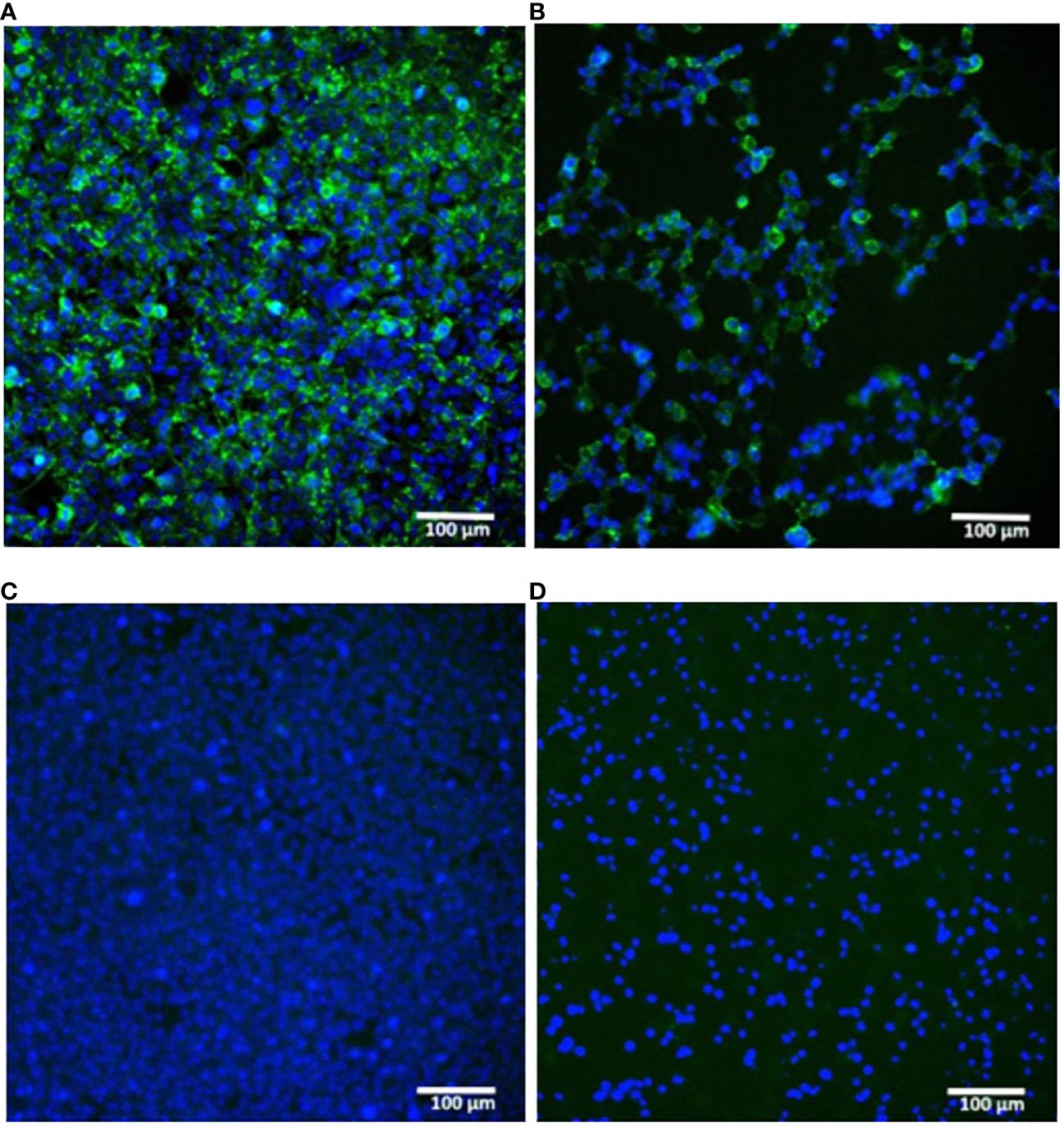
Figure 2 In vitro expression of hemagglutinin (H1N1 A/Wisconsin/588/2019) in HEK293 cells transfected with synthetic mRNA. (A, B) – mRNA-transfected HEK293 cells were immunostained for intracellular or surface HA protein respectively. (C, D) – the same staining of untransfected HEK293 cells (C) – intracellular, (D) – surface). Immunostaining was performed using primary polyclonal anti-Influenza A Antibody and Donkey Anti-Goat IgG H&L (Alexa Fluor ® 488) secondary Ab (green), nuclei were stained with DAPI (blue). Scale bar 100 µm.
These results confirmed the translational activity of our synthetic mRNAs in cultured cells. In particular, the presence of the HA antigen on the cell surface indicated the correct design of the mRNA coding part containing a region for the HA transmembrane domain, which ensures the anchoring of the antigen to the host cell membrane.
3.2 Immunogenicity of two doses of the multivalent HA-mRNA vaccine
Immunogenicity of prepared mRNAs were investigated in mice using HAI assay, as described previously ( 29 ). Initially, we tested the immunogenicity of H1 and H3 HA-encoding mRNAs separately ( Supplementary Figure S1 ). HAI titers were measured after two 10-mg doses of individually formulated either H1 or H3 mRNA were administrated to BALB/c mice (n=6) intramuscularly (IM). HAI titers were up to 1:1280 above baseline by day 7 after second dose.
Next, to determine the immunogenicity of combined mRNA influenza vaccine candidate (mRNA-IV), HAI titers were determined in BALB/c mice (n=3) after two dose immunization with 15 µg of an equimolar mixture of individually formulated H1, H3, and IBV mRNA-LNPs ( Figure 3A ). Thus, one dose of mRNA-IV contains 5 µg of each mRNA. This dose was selected as a potential 1/10 human dose of mRNA, by analogy with vaccines for the prevention of COVID-19. One control group of mice (n=3) received 6 µg (1/10 human dose) of split inactivated influenza vaccine (SIIV), another control group (n=3) received equal volume of sterile PBS. The SIIV contained 4 antigens according to WHO recommendations for the 2022-2023 seasonal influenza vaccine in the northern hemisphere ( 28 ). The second dose was administered IM 14 days after the first dose.
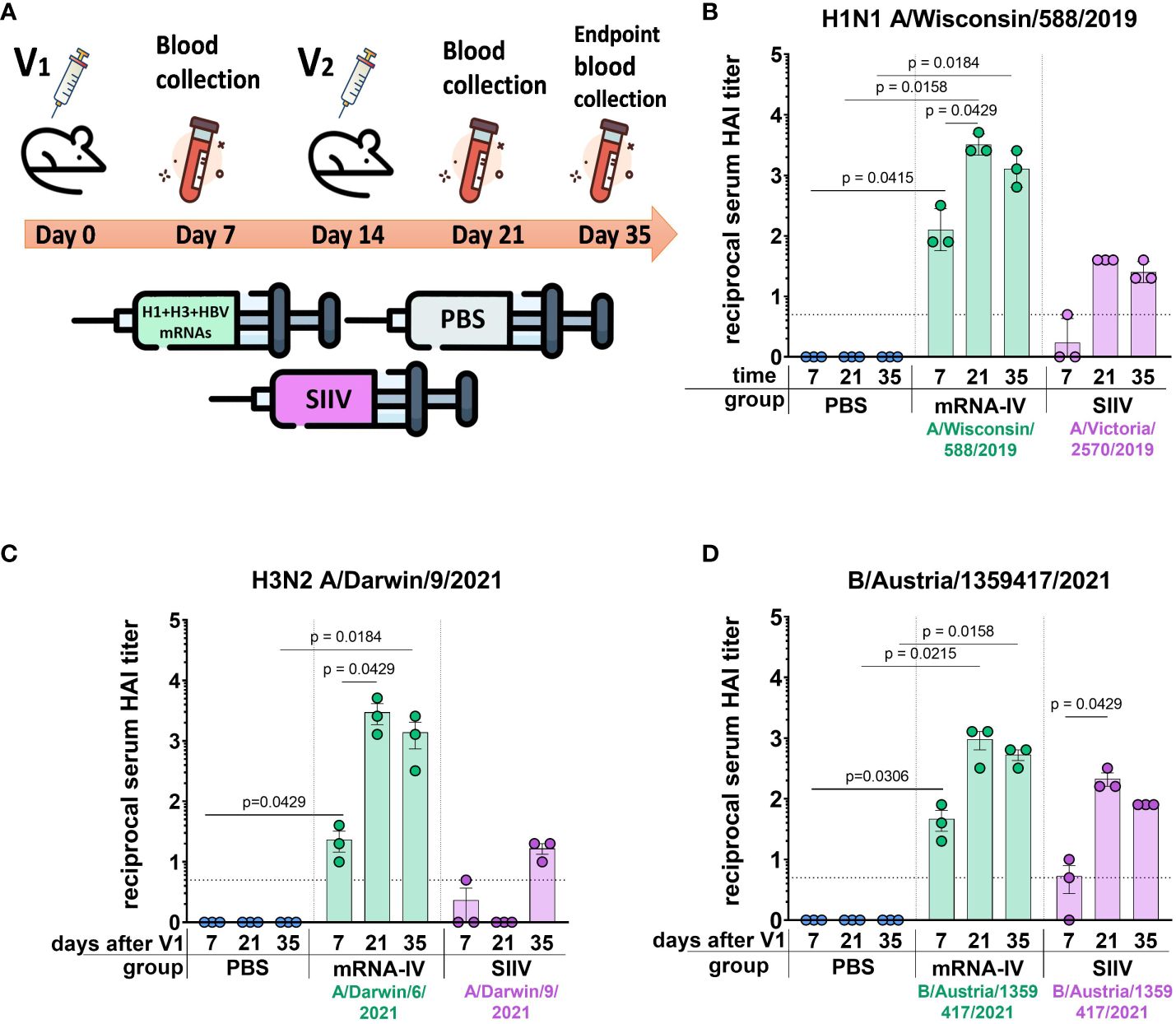
Figure 3 Mice immunized with mRNA-IV generate robust antibody responses. BALB/c mice (n=3 per group) were vaccinated intramuscularly at week 0 and 2 with a mixture of three different HA-mRNA-LNPs (a combined total dose of 15 µg mRNA per mouse (n=3), including 5 µg of each individual HA-mRNA-LNP). Control BALB/c mice were immunized intramuscularly with 1/10 human dose of quadrivalent split inactivated influenza vaccine (SIIV, a combined total dose of 6 µg per mouse, including 1.5 µg of each individual antigen), and another control BALB/c mice were administered PBS intramuscularly. (A) The scheme of the animal experiment. Serum HAI titers were determined after 7, 21, and 35 days post prime dose (V1) with antigens of vaccine strains: (B) H1N1 A/Wisconsin/588/2019, (C) – H3N2 A/Darwin/9/2021, (D) – B/Austria/1359417/2021. Group mRNA-IV shown in green, SIIV – in violet, and PBS – in blue. Data are representative of one experiment and shown as geometric means ± SD. Data were analyzed using a Kruskal-Wallys test with Dunn’s multiple comparison test for inter-group analysis and Friedman test with Dunn’s comparison test for intra-group analysis of HAI titers through the time.
3.2.1 H1 vaccine component
Analysis of the immune response to the H1 component of the mRNA vaccine showed high HAI titers against the homologous strain A/Wisconsin/588/2019 with geometric mean of 1:3413 (7 days after the second dose of vaccine). Compared to the control split vaccine (SIIV), the mRNA-LNP showed a rapid immune response significantly exceeding the limit of detection (LOD) as early as a week after the first vaccine dose. The average HAI titer against the A/Wisconsin/588/2019 antigen for the mRNA group after first dose was 1:126; for the SIIV group it was below the LOD (Mann-Whitney test p=0.1 , Figure 3B ). However, the Wilcoxon rank test showed no significant differences. On the 7th day after second vaccination, the differences in the level of antibody response in mRNA and split vaccine groups are especially noticeable - 1:3225 and 1:40, respectively (80-fold differences), however, due to the small sample size, significant differences between these groups could not be found. Also, if we consider changes in the level of immune response within the group over time (on days 7, 21, and 35 after V1), a significant increase in the immune response is noted for the mRNA group after the second vaccine dose. For the mRNA group HAI titers against H1N1 on day 21 after V1 significantly exceed those on day 7 after V1 ( p=0.0429 , Friedman multiple comparison test). A decrease in antibody response by day 35 after V1 compared to day 21 was statistically not significant (Wilcoxon test p=0.25 ), the mean HAI titers differ by 2.2 times ( Figure 3B ). These differences are within the error of the HAI method, since the serum dilution factor in the study was equal to 2. A similar comparison in the SIIV group also did not reveal significant differences in HAI titers between 21 and 35 days against the A/Wisconsin/588/2019 strain ( p=0.75 ). This may be due to the small sample size.
3.2.2 H3 vaccine component
For the H3 component of the vaccines, we obtained a similar immunological profile, but with more pronounced differences between mRNA and split vaccine in terms of the level of immune response ( Figure 3C ). As we did not have the influenza vaccine strain A/Darwin/9/2021 in our virus strain collection, HAI titers were determined using the antigen of serologically close strain A/Darwin/6/2021 (2 amino acids substitutions in HA protein sequence - G69D, D202N). Thus, H3-mRNA (A/Darwin/9/2021), in trivalent mRNA-IV vaccine caused a detectable antibody response already on day 7 after V1 (mean 1:23), while for split vaccine this value was below the LOD ( Figure 3C ). Seven days after the second vaccination, the mean titer of HAI in the mRNA group reaches 1:2987, while in sera from mice that received the split inactivated vaccine, this value remains below the LOD ( Figure 3C ). Increase of HAI titer in serum of mice vaccinated by SIIV was observed only to 35 days after V1, however it remains 86-fold lower than this value for the mRNA vaccine (mean HAI titer in mRNA vaccinated mice - 1:1387). When comparing immunogenicity through the time of experiment (7, 21, and 35 days after V1) within the mRNA group, there was observed significant increase after the second dose of mRNA on day 21 (with a mean titer of 1:2987), in comparison with serum titers on day 7 ( p=0.0429 , Friedman multiple comparison test). There were no significant differences in titers between days 21 and 35 ( p=0.66 ).
3.2.3 B (Victoria) vaccine component
The immune response in vaccinated mice to influenza B virus (Victoria lineage) was lower compared to two previous vaccine components (H1 and H3). Two-dose vaccination with mRNA-LNP resulted in lower serum HAI titers to B/Austria/1359417/2021 antigens than to H1N1 antigens (mean HAI titer in mRNA vaccinated mice – 1:960 at 21 days post V1, Figure 3D ). However, just 7 days after the first dose, HAI titers for the mRNA group exceeded the LOD (mean HAI titer - 1:45), while in the split vaccine group, the mean HAI titer was below the LOD (mean HAI titer - 1:5). The difference in the level of immune response to mRNA and split vaccines is much less for the B component of the vaccine than for the H1 and H3 components. A decrease in antibody response by day 35 after V1 compared to day 21 was not statistically significant ( p=0.25 , Wilcoxon test).
3.2.4 Cross-reactivity of immune response 3 weeks after two-dose vaccination
On day 35 after the start of the experiment, the whole blood was collected from the mice for more in-depth studies. This allowed us to study the cross-reactivity of the immune response after the vaccination to more than one different influenza strain only for endpoint serum sample of mice (21 days post second dose, Figure 4 ). For the H1N1 strains we used (Wisconsin/588/2019, Victoria/2570/2021, Guangdong-Maonan/SWL1536/2019, Moscow/52/2022, California/07/2009), the immune response is highly cross-reactive in the group of mRNA-vaccinated animals. The geometric mean HAI titers against H1N1 strains were from 1:806 (against Guangdong-Maonan/SWL1536/2019 antigen) to 1:2032 ( Figure 4A ).
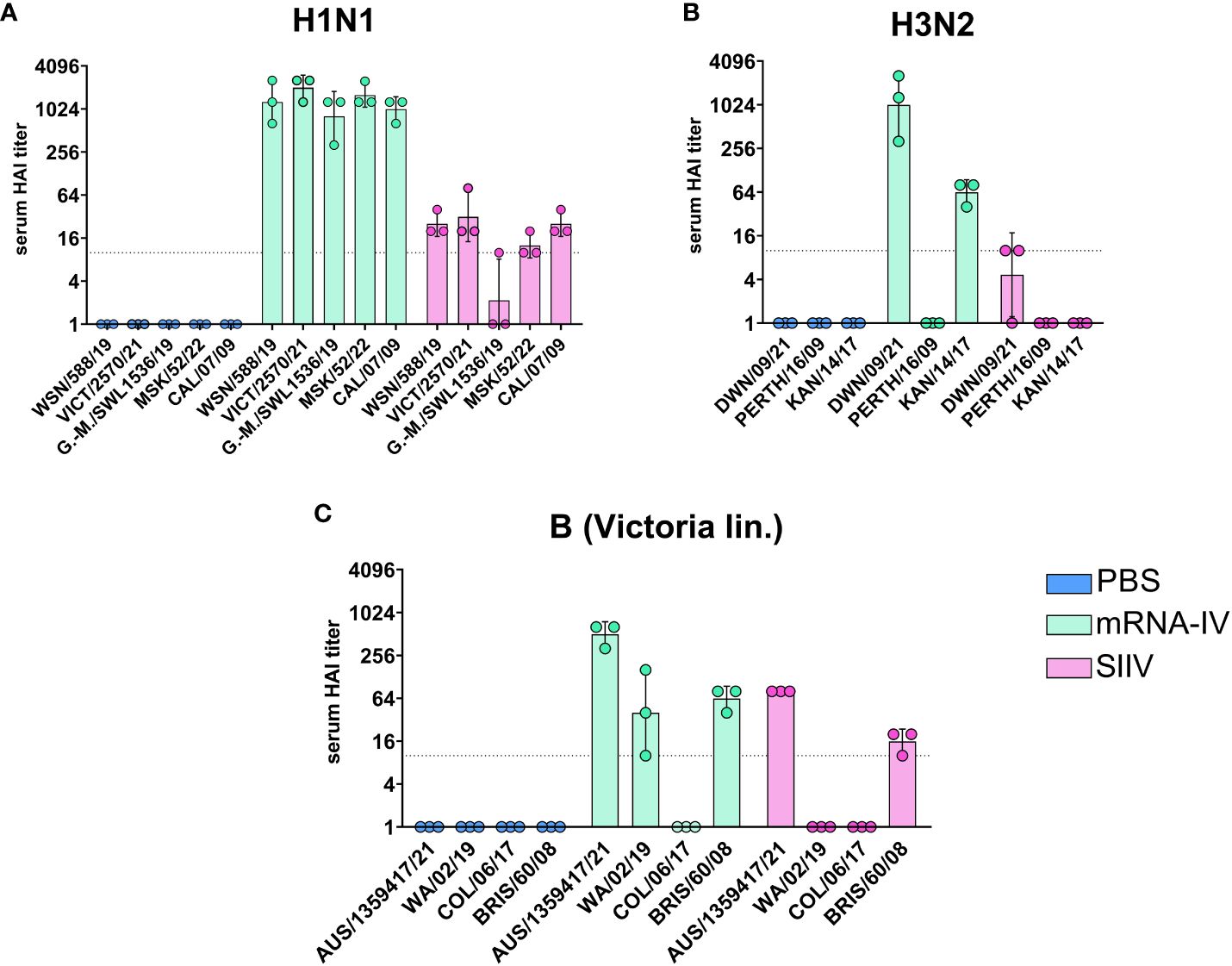
Figure 4 Mice immunized with mRNA-IV generate cross-reactive antibody responses. BALB/c mice (n=3 per group) were vaccinated intramuscularly at week 0 and 2 with 3 different HA mRNA-LNPs (a combined total dose of 15 µg per mouse (n=3), including 5 µg of each individual HA mRNA-LNP). Control BALB/c mice were immunized intramuscularly with 1/10 human dose of registered quadrivalent split inactivated influenza vaccine (SIIV, a combined total dose of 6 µg per mouse, containing 1.5 µg of each individual antigen), and another control BALB/c mice were administered PBS intramuscularly. Serum titers in heterologic HAI were determined after 35 days post prime dose with antigens of different influenza strains: (A) strains of H1N1 influenza subtype (Wisconsin/588/2019, Guangdong-Maonan/SWL1536/2019, Moscow/52/2022, California/07/2009), (B) – strains of H3N2 influenza subtype (Darwin/9/2021, Perth/165/2009, Kansas/14/2017), (C) – strains of influenza B (Victoria lineage – Austria/1359417/2021, Washington/02/2019, Colorado/06/2017, Brisbane/60/2008). Group mRNA-IV shown in green, SIIV - in violet and PBS - in blue. Data are representative of one experiment and are expressed as geometric means ± SD.
There was no significant reduction in HAI titers even to distant pandemic A/California/07/2009 strain with mean value 1:1015 ( Figure 4A ). The immune response to SIIV was not as robust as to mRNA-IV, the mean titer value did not exceed 1:31 (against Victoria/2570/2021 antigen), which more than 60-fold lower comparing with mRNA. For single H1-mRNA study of cross-reactivity of immune response showed similar results ( Supplementary Figure S2 ).
The cross-reactivity of immune response to H3 component ( Figure 4B ) of the vaccine was manifested by decreased titers to the Kansas/14/2017 strain (the geometric mean HAI titer was 1:63) and no detectable response to the Perth/165/2009 strain, while response to serologically close strain A/Darwin/6/2021 remained to be high with geometric mean HAI titer 1:1015.
Noticeable results were obtained in a heterologous HAI test with the antigens of influenza virus B strains (B/Washington/02/2019, Colorado/06/2017 and Brisbane/60/2008, Figure 3C ). Immune response against listed strains was reduced compared to homologous HAI results ( Figure 3C ) for both mRNA and split inactivated vaccine. In the mRNA group, the decrease in mean HAI titers to the Washington/02/2019 antigen was 12-fold and to the Brisbane/60/2008 antigen was 8-fold. No immune response to the Colorado/06/2017 antigen was detected in the serum of mice vaccinated with both mRNA and SIIV. In the SIIV group, no immune response to the Washington/02/2019 antigen was detected and the reduction in HAI titers to Brisbane/60/2008 compared to homologous was more than 5-fold.
We tested the hypothesis that the cross-reactivity of the immune response of mice after immunization with an mRNA vaccine is due to the high content of antibodies specific to the conservative domains of hemagglutinin by examining the sera of immunized mice in ELISA. The study involved sera received from mice (n=6 per group) 35 days post vaccination with trivalent mRNA, SIIV and PBS according to the previously described scheme ( Figure 3A ). ELISA analysis of sera was carried out with two antigens – a modified trimeric HA stem domain (influenza H1N1 A/Brisbane/59/2007) ( 27 ) and the HA head domain (HA1 subunit of influenza A H1N1 A/California/04/2009). Results of ELISAs demonstrated high antibody level against both HA subunits in mRNA vaccinated mice’s serum in comparison with ones vaccinated with SIIV ( Figures 5A–D ). Geometric mean of area under curve for mRNA group significantly differ from these values for PBS and SIIV groups in ELISA with both HA subunits ( Figures 5B, D ). The serum end-point titers, calculated based on ELISA data showed 100-fold and 1000-fold increase in antibody levels in mice vaccinated with mRNA compared to SIIV against HA head domain and HA stem domain respectively ( Supplementary Figures 3B, D ).
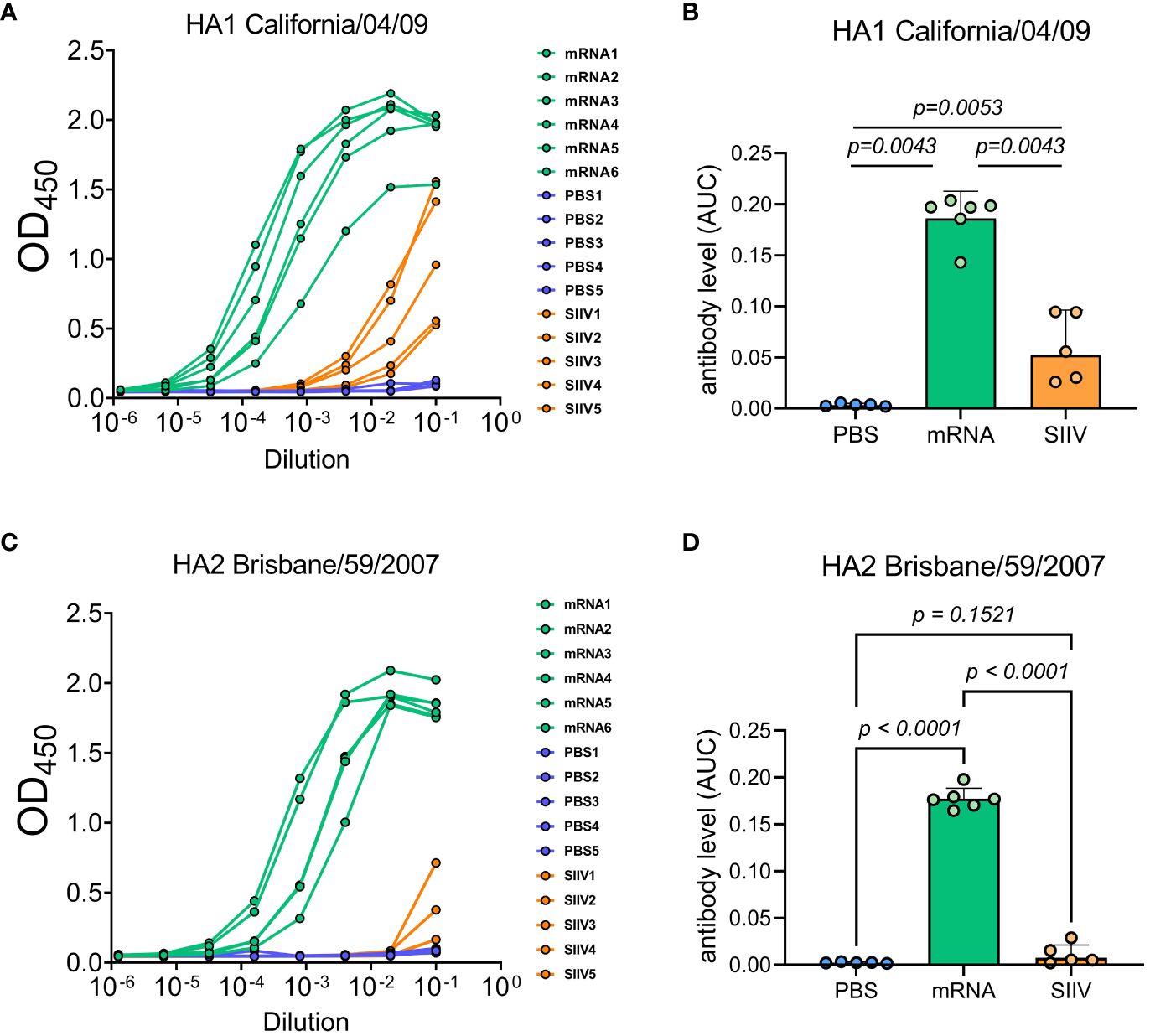
Figure 5 Antibody levels measured by ELISA to HA subunits of distant influenza A strains as possible explanation of cross-reactive immune response to the trivalent mRNA vaccination. Mice were twice vaccinated by trivalent mRNA, including 5 µg of each individual HA mRNA-LNP, or 1/10 human dose of SIIV, or PBS as earlier described. (A, C) Representative ELISA curves of antibody levels to HA head (HA1 subunit of influenza A H1N1 A/California/04/2009) and stem (modified trimeric HA2 influenza H1N1 A/Brisbane/59/2007) domains respectively. (B, D) The same data are represented as geometric means of area under ELISA curves and compared by Mann-Whitney test.
3.3 Protective efficacy of two doses of the trivalent HA-mRNA vaccine
In order to evaluate the effectiveness of immunity formed by mRNA-LNP vaccination, we conducted an experiment using an animal model of lethal influenza infection. This model included infection of BALB/c mice vaccinated according to the chosen regimen with mouse-adapted influenza A/Victoria/2570/2019 virus. The design of the experiment is shown in Figure 6A .
Animals immunized with PBS (n=13) and animals that received 1/10 human dose of inactivated split vaccine were used as comparison groups. Fourteen days after second immunization according to the scheme in Figure 6A mice were infected intranasally with 25 LD 50 of H1N1 pdm09 virus (A/Victoria/2570/2019, mouse adapted), which had 99.8% HA amino acid homology to the H1 component of the mRNA vaccine. On the third day after infection, 3 mice from each group were euthanized for lung isolation. Viral load was assessed in lung homogenates by RT-PCR with normalization to a housekeeping gene, PDK1 (encoding pyruvate dehydrogenase kinase-1). In the mRNA vaccinated mice group, the average viral load in the lungs was 3.5 copies/mL, while in the PBS and SIIV control groups, the mean Log10 viral load was 6.65 and 6.4 genome copies/mL, respectively ( Figure 6B ). The viral load in the lungs of mRNA vaccinated mice was almost undetectable in contrast to the PBS and SIIV control groups ( p<0.0001 and p=0.0382 , respectively, Figure 6B ). On day 6 post-infection, 46% of the animals in the control group died and all animals died on day 9 ( Figure 6C ). In the group of animals that received the inactivated split vaccine, the lethality rate on (or between) days 7–9 of infection was 23%. No animal deaths were observed in the group that received the mRNA-IV. Both vaccines protected animals from 25 LD 50 doses of virus with statistical reliability ( p<0.0001 compared to the PBS group). No significant differences were found between vaccines according to these statistical tests. Evaluation of weight dynamics shows that animals from the control group started active weight loss from the second day after infection ( Figure 6D ). In the group of animals receiving SIIV, weight loss was observed until the 3rd day of infection with subsequent recovery. No weight loss was observed in the group of animals receiving mRNA. On day 1 after infection, an average increase in body weight of 3% was observed for animals in the SIIV group and the value of this parameter was significantly higher than that of the mRNA-IV group. However, from day 2 post-infection until the end of observations (day 10), weight loss was minimal for the mRNA-IV group and was significantly lower for the other two groups of mice that received inactivated vaccine or PBS ( Figure 6D ).
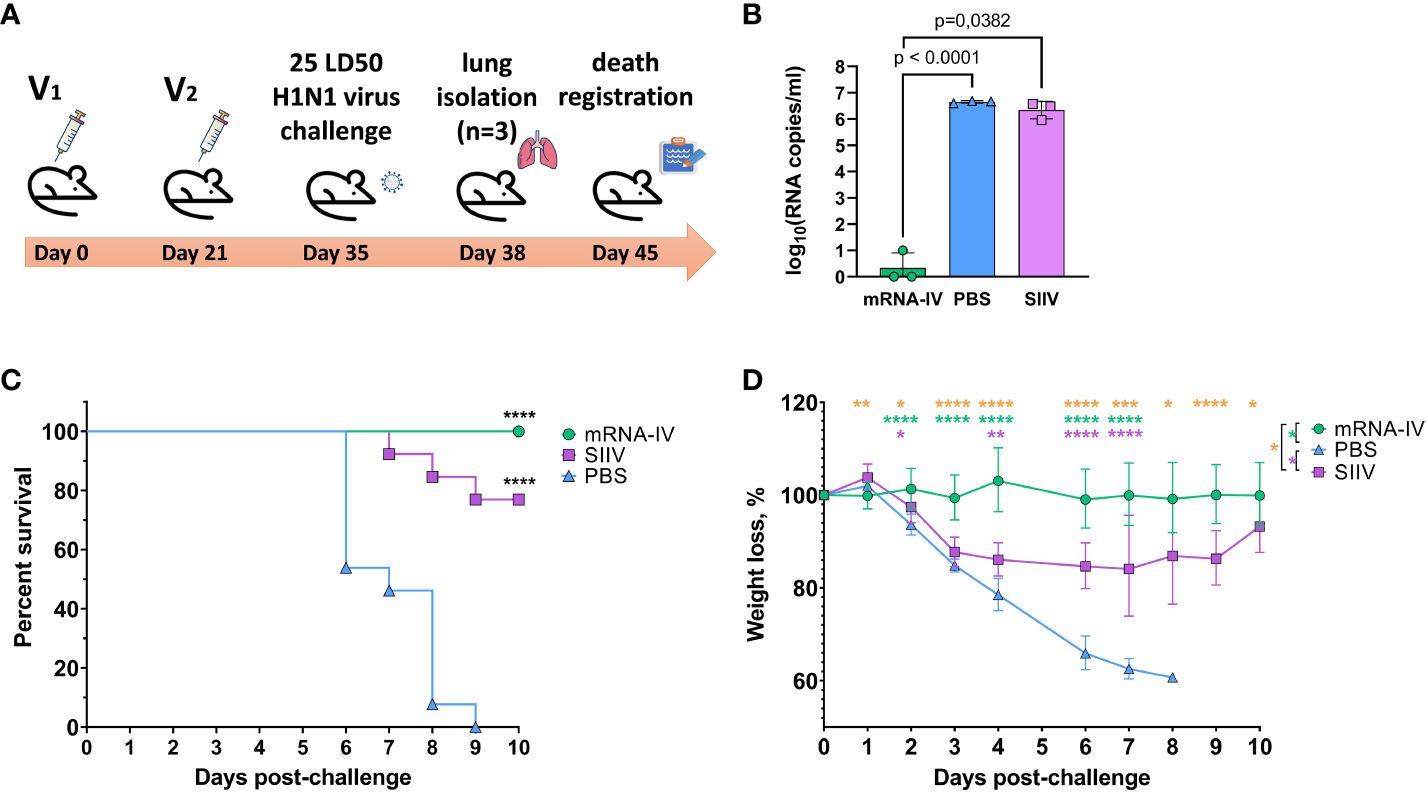
Figure 6 The mRNA-IV vaccine protects mice from influenza infection with antigenically close H1N1 strain. BALB/c mice were immunized with two-dose of mRNA vaccine and challenged with 25 LD 50 of H1N1 virus (A/Victoria/2570/2019, mouse adapted). (A) The scheme of challenge experiment. At third day post infection lungs of mice (n=3) from every group were harvested and analyzed by qPCR for viral load determination (B) . Survival (C) and weight loss (D) of the remaining animals were recorded post challenge. Data are representative of one experiment and shown as geometric means ± SD. Data were compared using a Long-rank (Mantel-Cox) test with Dunn’s multiple comparison test for survival analysis and Tukey’s multiple comparisons test for analysis of weight loss differences between groups. The viral load data were compared using Mann-Whitney test. P-value shown as asterisks, *p< 0.05, **p< 0.01, ***p< 0.001, ****p< 0.0001 .
4 Discussion
Due to the ongoing genetic perturbations and evolution of influenza viruses, including antigenic drift ( 30 ) and recombination ( 31 , 32 ), the antigenic characteristics of the pathogen undergo continuous changes. As a result, the immunity generated by previous exposure or vaccination becomes less effective. This continually puts the human population at risk of seasonal influenza epidemics. To address this challenge, the WHO Global Influenza Surveillance and Response System (GISRS) monitors influenza viruses circulating in humans and updates the antigenic composition of vaccines twice a year (for the southern and northern hemispheres) ( 33 ). Manufacturers of influenza vaccines receive guidance on the specific antigenic composition, including two serologic variants of influenza A and two serologic variants of influenza B, to be incorporated in vaccines for the upcoming season ( 34 ). However, mistakes in the strain selection, as well as the need to enhance protection in vulnerable groups, have prompted the search for novel approaches to develop influenza vaccines that offer broader and more effective protection ( 35 , 36 ).
The mRNA platforms hold significant promise for the development of influenza vaccines and offer several advantages. First, it enables rapid vaccine creation and production in event of a completely new virus strain, allowing for a timelier response to emerging threats ( 37 ). Second, unlike traditional methods, mRNA vaccine production does not require the use of live virus and can be based on synthetic sequences obtained in the laboratory. This eliminates the risk of unintended mutations that may arise from virus passages. Third, mRNA-based vaccines are highly precise in targeting the immune response as they express only specific antigens, such as influenza HA ( 38 ). Finally, the delivery of mRNA formulated in LNPs not only promotes both humoral and cellular immune responses but also activates the innate immune system, further enhancing the effectiveness of the vaccine ( 39 ).
In our work, we present the results of creating a candidate mRNA vaccine with an antigen composition recommended by the WHO for use in seasonal vaccines in the northern hemisphere in 2022-2023: A/Wisconsin/588/2019 (H1), A/Darwin/6/2021 (H3), and B/Austria/1359417/2021 (IBV, Victoria lineage) ( 28 ). We hypothesized that the cross-reactivity of the immune response toward heterologous strains of the influenza virus can be obtained due to the features of mRNA vaccine platform. The viral antigen is synthesized directly in the cells of the immunized organism, with a natural folding and glycosylation pattern. It undergoes processing in proteasomes and is displayed by MHC class I proteins. Additionally, antigens that are secreted can be taken up by cells, degraded inside endosomes, and presented on the cell surface to helper T cells by MHC class II proteins ( 37 ). We used a quadrivalent inactivated split vaccine as a control, which also has the recommended antigen composition. In the main series of experiments, we used vaccines at 1/10 of the human dose (set in the case of split vaccine and assumed for the mRNA vaccine) to allow comparison of platforms when assessing immunogenicity in mice. Both vaccines showed detected immunogenicity using the HAI method. This method is the gold standard for assessing the immunogenicity of influenza vaccines and the efficacy of immunity against novel virus variants ( 29 ).
The level of humoral response in HAI to the mRNA vaccine injection was on average 10-100 times higher than that to the split inactivated vaccine for all subtypes. This correlates with previously obtained data for the combined mRNA vaccine ( 40 ). When immunogenicity was analyzed by ELISA method (against H1N1pdm) for the group of mice immunized with the combined mRNA vaccine, as well as in our case, 10-100 times higher levels of antibodies (depending on the dose of mRNA) were observed compared to this value in the group of animals vaccinated with quadrivalent inactivated vaccine (at a dose of 1.5 µg). The levels of antibody response for the inactivated vaccine obtained in our experiments were in good agreement with the data of the cell-based split vaccine study ( 41 ). For a 1.5 μg dose of each antigen separately, HAI titers comparable to those obtained in our work for the quadrivalent vaccine were obtained (HAI titers to H1N1 averaged about 1:90, to H3N2 averaged about 1:500, and to B (Victoria lineage) averaged about 1:30). One could speculate that this difference between the immune response to the mRNA and the split vaccine could be due to the dose of antigen delivered. Thus, in the case of the inactivated vaccine, the dose of individual antigens was 1.5 μg, whereas for the mRNA composite, the dose of each antigen was 5 μg. Unfortunately, there is no adequate method to quantitatively compare antigen delivered using different vaccine platforms. Delivery of the antigenic protein and its analogue in mRNA form initially yields different pharmacokinetics. According to one of the studies, based on the results of an in vitro experiment, 1 pmol of the spike protein was obtained using 6 pmol of mRNA vaccine for 24 hours during transfection of BHK cell culture ( 42 ). According to our earlier experiments, when we delivered 10 μg of mRNA (approximately 20 pmol) encoding the B11 antibody against botulinum toxin we achieved a maximum antibody concentration in mouse serum of 99 ng/mL, which translates to about 2.35 pmol of antibody per average serum volume of an 18-g mouse ( 21 ). Both examples indicate that the amount of antigen produced with the target mRNA is 5-10 times less compared to the amount of mRNA used. Thus, it is more likely that the advantage of mRNA in the context of the generated humoral response is not due to the obviously higher concentration of antigen achieved by the selected dos-es, but is due to a complex of differences including the mRNA platform itself compared to the delivery of purified antigens used in split vaccines.
As a result of the protective efficacy study using 25 LD 50 of H1N1 virus (A/Victoria/2570/2019, mouse adapted), we demonstrated the protective effect of both mRNA and split vaccine compositions. In the case of mRNA, no animal deaths were registered during the observation period, whereas in the group of mice that received split vaccine, 3 out of 10 animals died. These data correlated with the dynamics of weight of infected animals (for split vaccine, weight loss was observed from days 3 to 9 of observation), as well as viral load in the lungs - on day 3, the difference in viral load between the groups that received mRNA composition and split vaccine was 10 6 . We were unable to find studies that examined the protectiveness of mRNA and split vaccines in a single animal experiment, but there are studies of split vaccine candidates produced by virus production in the MDCK cell line ( 41 ). On day 4 after 10 7 cell culture infectious dose 50% (CCID 50 ) infection with H1N1 influenza virus, a weight loss of up to 10% was observed in the group of mice vaccinated with 5 μg split mono-vaccine, and the amount of virus in the lungs of infected mice isolated on day 6 was 10 2 virus copies/mL, compared with 10 5 virus copies/mL in the placebo group. This is indicative that both in our case and in the Zhang et al. study, influenza virus was detected by qPCR in the lungs of animals vaccinated with inactivated split vaccines after infection. In our study, in the mRNA group, the viral load in the lungs was below the detection limit, which is consistent with the data obtained by Arevalo et al. for both 20-valent and monovalent H1-mRNA influenza vaccines ( 16 ). In the lungs of control mice immunized with mRNA vector with luciferase (placebo), the viral load determined by median tissue culture infectious dose 50% (TCID 50 ) assay was approximately 5×10 4 TCID 50 /mL, and in groups of mice immunized with mono H1-mRNA preparation or 20-valent mRNA, the viral load was below the detection limit. As for the results of animal death in the split vaccine group (n = 3), this effect was observed by researchers of adjuvants for influenza vaccines ( 43 ). When intranasally administering a split vaccine, they observed 100% mortality of animals by day 6 after infection with 10 LD 50 adapted to mice influenza virus A H1N1 A/California/04/2009. On the 3rd day after infection, the virus titer in the bronchoalveolar lavage of infected mice was 6.7 ± 0.1 Log10 PFU/mL. In general, the death of mice immunized with split influenza vaccines after infection with different doses of influenza viruses is not a new phenomenon. It has been noted in other studies as well ( 43 , 44 ). Our results for the split-vaccine in terms of the efficacy of protecting vaccinated mice from death are consistent with the published data. However, for preclinical studies of influenza vaccine efficacy, ferrets are the preferred animal model ( 45 ).
As a result of using heterologous to the vaccine influenza virus antigens in the HAI test, we demonstrated a broader serum hemagglutinating activity in mice receiving the mRNA composition compared to those receiving the split inactivated influenza vaccine. The immune response to the B/Washington/02/219 strain, which was heterologous to the vaccine strain, was at a level exceeding the lower limit of detection and increased after repeated vaccination. However, in the group of animals immunized with the split vaccine, serum HAI titers were below the LOD at all-time points, and no enhancement of immunity by repeated vaccination was observed. The findings indicate a higher potential for the formation of a cross-specific humoral immune response by mRNA compositions. An extended study with four different H1N1 antigens confirmed the findings. Statistically significant differences in serum activity against evolutionarily more distant virus variants (A/California/07/2009pmd, A/Guangdong-Maonan/SWL1536/2019), especially on day 14 after immunization, were detected. However, on day 39, the sera showed high activity against all variants; differences in the level of immune response in hetero- and homologous HAI titers were statistically insignificant, and average serum titers ranged from 1:160 (in the case of the most distant A/California/07/2009pdm) to 1:1280 (in the case of the epidemic strain A/Moscow/52/22, which circulated in the epidemic season 2022–23 after the vaccine was released). These data correlate with the results of previously published works, which demonstrated the presence of binding and neutralizing antibodies against the H1N1 strain (A/Michigan/45/2015, distant to the vaccine) in the monovalent mRNA vaccine. However, a two-fold reduction in the binding level and the absence of neutralizing antibodies were observed against the second, more distant H1N1 strain, A/Puerto-Rico/08/1934 [16]. We have demonstrated the cross-immunity due to mRNA vaccination against a strain that emerged after updating the vaccine composition for the 2022-2023 season, acquiring 9 substitutions in the amino acid sequence of HA (relative to A/Wisconsin/588/2019). It is important to note that cross-reactivity of the immune response to mRNA vaccine is achieved by the presence of HA mRNA from one strain of influenza subtype in the vaccine.
We hypothesized that the cross-reactivity of the immune response after immunization with an mRNA vaccine is due to the higher content of antibodies specific to the conservative domains of hemagglutinin. Our hypothesis was supported by our results of antibody levels estimation to HA stem domain. The serum end-point titers were 1000-fold higher in mice vaccinated with mRNA compared to SIIV. A recombinant modified trimeric HA stem domain (#4900) has well known as broadly protective immunogen ( 46 ) and due to modifications, it has low identity in amino acid sequence with the stem domain of mRNA coding HA and HA from SIIV (78.9% both). As for the head HA subunit serum end-point titers was a 100-fold higher in mice vaccinated with mRNA compared to SIIV. It can additionally provide quantitative and qualitative advantage increasing cross-reactivity of the immune response. It is known, that head domain of HA has some conservative regions, which are have been showed to be a target for broad neutralizing antibodies ( 47 – 49 ). Thus, our results suggest that the nature of the broader immune response may be due to qualitative and quantitative advantages of antibodies generated by mRNA vaccines compared to split vaccines. In turn, this may be related to the ability of the platform mRNA to provide the natural folding and glycosylation pattern of the antigen.
In summary, the results presented in this study highlight the potential of mRNA-based platforms for the development of influenza vaccines and suggest the need for further research in this area. To fully understand the universality, breadth, and effectiveness of the immune response elicited by mRNA vaccines, it is crucial to conduct additional studies evaluating the immunogenicity and protective efficacy against more diverse strains of H1, H3, and BV in vivo . It is also important to validate the data obtained from hemagglutination tests by conducting sera microneutralization assays. Additionally, including a group of mice immunized with non-lethal doses of live virus as a positive control in immunogenicity studies can provide valuable insights into the models of the natural immunity. These findings hold significant implications for the development of mRNA vaccines that offer broader protection against a wide range of influenza strains.
Data availability statement
The raw data supporting the conclusions of this article will be made available by the authors, without undue reservation.
Ethics statement
The animal study was approved by The Institutional Animal Care and Use Committee (IACUC) of the Federal Research Centre of Epidemiology and Microbiology named after Honorary Academician N.F. Gamaleya. The study was conducted in accordance with the local legislation and institutional requirements.
Author contributions
EPM: Writing – original draft, Methodology, Investigation, Formal analysis, Data curation, Conceptualization. VG: Writing – original draft, Supervision, Project administration, Investigation, Conceptualization. DK: Writing – review & editing, Investigation. AS: Writing – review & editing, Methodology, Investigation. EIB: Writing – review & editing, Supervision, Methodology. MS: Writing – review & editing, Resources, Investigation. EAM: Writing – review & editing, Investigation, Formal analysis. ENB: Writing – review & editing, Investigation. SK: Writing – review & editing, Investigation, Formal analysis. EE: Writing – review & editing, Resources, Investigation. AZ: Writing – review & editing, Investigation. ES: Writing – review & editing, Investigation. EK: Writing – review & editing, Investigation. AK: Writing – review & editing, Investigation. EU: Writing – review & editing, Investigation. NK: Writing – review & editing, Investigation. II: Writing – review & editing, Resources, Investigation. SD: Writing – review & editing. RI: Writing – review & editing. DL: Writing – review & editing, Supervision, Investigation. AG: Writing – review & editing, Supervision.
The author(s) declare financial support was received for the research, authorship, and/or publication of this article. This research was funded by National Research Centre for Epidemiology and Microbiology named after Honorary Academician N F Gamaleya (from the income-generating activities) and the grant #121102500071-6 provided by the Ministry of Health of the Russian Federation, Russia.
Acknowledgments
We are grateful to Dr. Dmitry V. Shcheblyakov and Daria V. Voronina from the laboratory of immunobiotechnology of the National Research Centre for Epidemiology and Microbiology Named after Honorary Academician N. F. Gamaleya for providing ELISA proteins.
Conflict of interest
The authors declare that the research was conducted in the absence of any commercial or financial relationships that could be construed as a potential conflict of interest.
Publisher’s note
All claims expressed in this article are solely those of the authors and do not necessarily represent those of their affiliated organizations, or those of the publisher, the editors and the reviewers. Any product that may be evaluated in this article, or claim that may be made by its manufacturer, is not guaranteed or endorsed by the publisher.
Supplementary material
The Supplementary Material for this article can be found online at: https://www.frontiersin.org/articles/10.3389/fimmu.2024.1381508/full#supplementary-material
1. Krammer F, Smith GJD, Fouchier RAM, Peiris M, Kedzierska K, Doherty PC, et al. Influenza. Nat Rev Dis Primers . (2018) 4:3. doi: 10.1038/s41572-018-0002-y
PubMed Abstract | CrossRef Full Text | Google Scholar
2. Factsheet about seasonal influenza (2017). Available online at: https://www.ecdc.europa.eu/en/seasonal-influenza/facts/factsheet (Accessed December 13, 2023).
Google Scholar
3. CDC. People at High Risk of Flu (2023). Centers for Disease Control and Prevention. Available online at: https://www.cdc.gov/flu/highrisk/index.htm (Accessed December 13, 2023).
4. Troeger CE, Blacker BF, Khalil IA, Zimsen SRM, Albertson SB, Abate D, et al. Mortality, morbidity, and hospitalisations due to influenza lower respiratory tract infections, 2017: an analysis for the Global Burden of Disease Study 2017. Lancet Respir Med . (2019) 7:69–89. doi: 10.1016/S2213-2600(18)30496-X
5. Sekiya T, Ohno M, Nomura N, Handabile C, Shingai M, Jackson DC, et al. Selecting and using the appropriate influenza vaccine for each individual. Viruses . (2021) 13:971. doi: 10.3390/v13060971
6. Tenforde MW, Kondor RJG, Chung JR, Zimmerman RK, Nowalk MP, Jackson ML, et al. Effect of antigenic drift on influenza vaccine effectiveness in the United States—2019–2020. Clin Infect Dis . (2021) 73:e4244–50. doi: 10.1093/cid/ciaa1884
7. Tanner AR, Dorey RB, Brendish NJ, Clark TW. Influenza vaccination: protecting the most vulnerable. Eur Respir Rev . (2021) 30:200258. doi: 10.1183/16000617.0258-2020
8. CDC. Key Facts About Seasonal Flu Vaccine (2023). Centers for Disease Control and Prevention. Available online at: https://t.cdc.gov/2S4F (Accessed December 13, 2023).
9. Ferdinands JM, Thompson MG, Blanton L, Spencer S, Grant L, Fry AM. Does influenza vaccination attenuate the severity of breakthrough infections? A narrative review and recommendations for further research. Vaccine . (2021) 39:3678–95. doi: 10.1016/j.vaccine.2021.05.011
10. Ferdinands JM, Olsho LEW, Agan AA, Bhat N, Sullivan RM, Hall M, et al. Effectiveness of influenza vaccine against life-threatening RT-PCR-confirmed influenza illness in US children, 2010–2012. J Infect Dis . (2014) 210:674–83. doi: 10.1093/infdis/jiu185
11. Rondy M, El Omeiri N, Thompson MG, Levêque A, Moren A, Sullivan SG. Effectiveness of influenza vaccines in preventing severe influenza illness among adults: A systematic review and meta-analysis of test-negative design case-control studies. J Infect . (2017) 75:381–94. doi: 10.1016/j.jinf.2017.09.010
12. Xie H, Wan X-F, Ye Z, Plant EP, Zhao Y, Xu Y, et al. H3N2 mismatch of 2014–15 northern hemisphere influenza vaccines and head-to-head comparison between human and ferret antisera derived antigenic maps. Sci Rep . (2015) 5:15279. doi: 10.1038/srep15279
13. Erbelding EJ, Post DJ, Stemmy EJ, Roberts PC, Augustine AD, Ferguson S, et al. A universal influenza vaccine: the strategic plan for the national institute of allergy and infectious diseases. J Infect Dis . (2018) 218:347–54. doi: 10.1093/infdis/jiy103
14. Polack FP, Thomas SJ, Kitchin N, Absalon J, Gurtman A, Lockhart S, et al. Safety and efficacy of the BNT162b2 mRNA covid-19 vaccine. N Engl J Med . (2020) 383:2603–15. doi: 10.1056/NEJMoa2034577
15. Baden LR, El Sahly HM, Essink B, Kotloff K, Frey S, Novak R, et al. Efficacy and safety of the mRNA-1273 SARS-coV-2 vaccine. N Engl J Med . (2021) 384:403–16. doi: 10.1056/NEJMoa2035389
16. Arevalo CP, Bolton MJ, Le Sage V, Ye N, Furey C, Muramatsu H, et al. A multivalent nucleoside-modified mRNA vaccine against all known influenza virus subtypes. Science . (2022) 378:899–904. doi: 10.1126/science.abm0271
17. Nachbagauer R, Liu W-C, Choi A, Wohlbold TJ, Atlas T, Rajendran M, et al. A universal influenza virus vaccine candidate confers protection against pandemic H1N1 infection in preclinical ferret studies. NPJ Vaccines . (2017) 2:26. doi: 10.1038/s41541-017-0026-4
18. Pardi N, Parkhouse K, Kirkpatrick E, McMahon M, Zost SJ, Mui BL, et al. Nucleoside-modified mRNA immunization elicits influenza virus hemagglutinin stalk-specific antibodies. Nat Commun . (2018) 9:3361. doi: 10.1038/s41467-018-05482-0
19. Bahl K, Senn JJ, Yuzhakov O, Bulychev A, Brito LA, Hassett KJ, et al. Preclinical and Clinical Demonstration of Immunogenicity by mRNA Vaccines against H10N8 and H7N9 Influenza Viruses. Mol Ther . (2017) 25:1316–27. doi: 10.1016/j.ymthe.2017.03.035
20. Feldman RA, Fuhr R, Smolenov I, Ribeiro A, Panther L, Watson M, et al. mRNA vaccines against H10N8 and H7N9 influenza viruses of pandemic potential are immunogenic and well tolerated in healthy adults in phase 1 randomized clinical trials. Vaccine . (2019) 37:3326–34. doi: 10.1016/j.vaccine.2019.04.074
21. Panova EA, Kleymenov DA, Shcheblyakov DV, Bykonia EN, Mazunina EP, Dzharullaeva AS, et al. Single-domain antibody delivery using an mRNA platform protects against lethal doses of botulinum neurotoxin A. Front Immunol . (2023) 14:1098302. doi: 10.3389/fimmu.2023.1098302
22. Brauer R, Chen P. Influenza virus propagation in embryonated chicken eggs. J Vis Exp . (2015) 97:e52421. doi: 10.3791/52421
CrossRef Full Text | Google Scholar
23. Bazarragchaa E, Hiono T, Isoda N, Hayashi H, Okamatsu M, Sakoda Y. Establishment of a mouse- and egg-adapted strain for the evaluation of vaccine potency against H3N2 variant influenza virus in mice. J Vet Med Sci . (2021) 83:1694–701. doi: 10.1292/jvms.21-0350
24. FELASA working group on revision of guidelines for health monitoring of rodents and rabbits, Mähler Convenor M, Berard M, Feinstein R, Gallagher A, Illgen-Wilcke B, et al. FELASA recommendations for the health monitoring of mouse, rat, hamster, Guinea pig and rabbit colonies in breeding and experimental units. Lab Anim . (2014) 48:178–92. doi: 10.1177/0023677213516312
25. ГОСТ 33044-2014 (2015). Available online at: https://docs.cntd.ru/document/1200115791 (Accessed December 13, 2023).
26. World Health Organization. Manual for the laboratory diagnosis and virological surveillance of influenza, in: WHO global influenza surveillance network: manual for the laboratory diagnosis and virological surveillance of influenza (2011). Available online at: https://iris.who.int/handle/10665/44518 (Accessed January 22, 2024).
27. Voronina DV, Shcheblyakov DV, Esmagambetov IB, Derkaev AA, Popova O, Shcherbinin DN. Development of neutralizing nanobodies to the hemagglutinin stem domain of influenza A viruses. Acta Naturae . (2021) 13:33–41. doi: 10.32607/actanaturae.11495
28. Recommendations announced for influenza vaccine composition for the 2022-2023 northern hemisphere influenza season. Available online at: https://www.who.int/news/item/25-02-2022-recommendations-announced-for-influenza-vaccine-composition-for-the-2022-2023-northern-hemisphere-influenza-season .
29. L’vov DK, Burtseva EI, Kolobukhina LV, Fedyakina IT, Bovin NV, Ignatjeva AV, et al. Peculiarities of the influenza and ARVI viruses circulation during epidemic season 2019–2020 in some regions of Russia. Prob Virol . (2021) 65:335–49. doi: 10.36233/0507-4088-2020-65-6-4
30. Both GW, Sleigh MJ, Cox NJ, Kendal AP. Antigenic drift in influenza virus H3 hemagglutinin from 1968 to 1980: multiple evolutionary pathways and sequential amino acid changes at key antigenic sites. J Virol . (1983) 48:52–60. doi: 10.1128/jvi.48.1.52-60.1983
31. Hirst GK, Gotlieb T. THE EXPERIMENTAL PRODUCTION OF COMBINATION FORMS OF VIRUS. J Exp Med . (1953) 98:41–52. doi: 10.1084/jem.98.1.41
32. Gibbs MJ, Armstrong JS, Gibbs AJ. Recombination in the hemagglutinin gene of the 1918 “Spanish flu”. Science . (2001) 293:1842–5. doi: 10.1126/science.1061662
33. Influenza (Seasonal) (2023). Available online at: https://www.who.int/news-room/fact-sheets/detail/influenza-(seasonal) (Accessed December 13, 2023).
34. Recommendations for influenza vaccine composition (2023). WHO. Available at: https://www.who.int/teams/global-influenza-programme/vaccines/who-recommendations (Accessed December 13, 2023).
35. Krammer F. The human antibody response to influenza A virus infection and vaccination. Nat Rev Immunol . (2019) 19:383–97. doi: 10.1038/s41577-019-0143-6
36. Ueno H. Tfh cell response in influenza vaccines in humans: what is visible and what is invisible. Curr Opin Immunol . (2019) 59:9–14. doi: 10.1016/j.coi.2019.02.007
37. Chaudhary N, Weissman D, Whitehead KA. mRNA vaccines for infectious diseases: principles, delivery and clinical translation. Nat Rev Drug Discov . (2021) 20:817–38. doi: 10.1038/s41573-021-00283-5
38. Wong S-S, Webby RJ. Traditional and new influenza vaccines. Clin Microbiol Rev . (2013) 26:476–92. doi: 10.1128/CMR.00097-12
39. Vogel AB, Kanevsky I, Che Y, Swanson KA, Muik A, Vormehr M, et al. BNT162b vaccines protect rhesus macaques from SARS-CoV-2. Nature . (2021) 592:283–9. doi: 10.1038/s41586-021-03275-y
40. Freyn AW, Ramos da Silva J, Rosado VC, Bliss CM, Pine M, Mui BL, et al. A multi-targeting, nucleoside-modified mRNA influenza virus vaccine provides broad protection in mice. Mol Ther . (2020) 28:1569–84. doi: 10.1016/j.ymthe.2020.04.018
41. Zhang J, Nian X, Liu B, Zhang Z, Zhao W, Han X, et al. Development of MDCK-based quadrivalent split seasonal influenza virus vaccine with high safety and immunoprotection: A preclinical study. Antiviral Res . (2023) 216:105639. doi: 10.1016/j.antiviral.2023.105639
42. Sutton WJH, Branham PJ, Williamson YM, Cooper HC, Najjar FN, Pierce-Ruiz CL, et al. Quantification of SARS-CoV-2 spike protein expression from mRNA vaccines using isotope dilution mass spectrometry. Vaccine . (2023) 41:3872–84. doi: 10.1016/j.vaccine.2023.04.044
43. van de Sandt CE, Kreijtz JHCM, Geelhoed-Mieras MM, Trierum SEV, Nieuwkoop NJ, van de Vijver DAMC, et al. Novel G3/DT adjuvant promotes the induction of protective T cells responses after vaccination with a seasonal trivalent inactivated split-virion influenza vaccine. Vaccine . (2014) 32:5614–23. doi: 10.1016/j.vaccine.2014.08.003
44. Boravleva EY, Lunitsin AV, Kaplun AP, Bykova NV, Krasilnikov IV, Gambaryan AS. Immune response and protective efficacy of inactivated and live influenza vaccines against homologous and heterosubtypic challenge. Biochem Moscow . (2020) 85:553–66. doi: 10.1134/S0006297920050041
45. Influenza vaccines - non-clinical and clinical module - Scientific guideline. European Medicines Agency. Available online at: https://www.ema.europa.eu/en/influenza-vaccines-non-clinical-and-clinical-module-scientific-guideline#ema-inpage-item-topics (Accessed December 13, 2023).
46. Impagliazzo A, Milder F, Kuipers H, Wagner MV, Zhu X, Hoffman RMB, et al. A stable trimeric influenza hemagglutinin stem as a broadly protective immunogen. Science . (2015) 349:1301–6. doi: 10.1126/science.aac7263
47. Bangaru S, Lang S, Schotsaert M, Vanderven HA, Zhu X, Kose N, et al. A site of vulnerability on the influenza virus hemagglutinin head domain trimer interface. Cell . (2019) 177:1136–1152.e18. doi: 10.1016/j.cell.2019.04.011
48. Whittle JRR, Zhang R, Khurana S, King LR, Manischewitz J, Golding H, et al. Broadly neutralizing human antibody that recognizes the receptor-binding pocket of influenza virus hemagglutinin. Proc Natl Acad Sci USA . (2011) 108:14216–21. doi: 10.1073/pnas.1111497108
49. Ekiert DC, Kashyap AK, Steel J, Rubrum A, Bhabha G, Khayat R, et al. Cross-neutralization of influenza A viruses mediated by a single antibody loop. Nature . (2012) 489:526–32. doi: 10.1038/nature11414
Keywords: seasonal influenza, vaccine, mRNA, immunogenicity, efficacy, immune response
Citation: Mazunina EP, Gushchin VA, Kleymenov DA, Siniavin AE, Burtseva EI, Shmarov MM, Mukasheva EA, Bykonia EN, Kozlova SR, Evgrafova EA, Zolotar AN, Shidlovskaya EV, Kirillova ES, Krepkaia AS, Usachev EV, Kuznetsova NA, Ivanov IA, Dmitriev SE, Ivanov RA, Logunov DY and Gintsburg AL (2024) Trivalent mRNA vaccine-candidate against seasonal flu with cross-specific humoral immune response. Front. Immunol. 15:1381508. doi: 10.3389/fimmu.2024.1381508
Received: 03 February 2024; Accepted: 29 March 2024; Published: 16 April 2024.
Reviewed by:
Copyright © 2024 Mazunina, Gushchin, Kleymenov, Siniavin, Burtseva, Shmarov, Mukasheva, Bykonia, Kozlova, Evgrafova, Zolotar, Shidlovskaya, Kirillova, Krepkaia, Usachev, Kuznetsova, Ivanov, Dmitriev, Ivanov, Logunov and Gintsburg. This is an open-access article distributed under the terms of the Creative Commons Attribution License (CC BY) . The use, distribution or reproduction in other forums is permitted, provided the original author(s) and the copyright owner(s) are credited and that the original publication in this journal is cited, in accordance with accepted academic practice. No use, distribution or reproduction is permitted which does not comply with these terms.
*Correspondence: Elena P. Mazunina, [email protected] ; Vladimir A. Gushchin, [email protected]
Disclaimer: All claims expressed in this article are solely those of the authors and do not necessarily represent those of their affiliated organizations, or those of the publisher, the editors and the reviewers. Any product that may be evaluated in this article or claim that may be made by its manufacturer is not guaranteed or endorsed by the publisher.
- Open access
- Published: 09 October 2021
Vaccination of older adults: Influenza, pneumococcal disease, herpes zoster, COVID-19 and beyond
- Birgit Weinberger ORCID: orcid.org/0000-0002-7084-946X 1
Immunity & Ageing volume 18 , Article number: 38 ( 2021 ) Cite this article
7117 Accesses
18 Citations
8 Altmetric
Metrics details
Preserving good health in old age is of utmost importance to alleviate societal, economic and health care-related challenges caused by an aging society. The prevalence and severity of many infectious diseases is higher in older adults, and in addition to the acute disease, long-term sequelae, such as exacerbation of underlying chronic disease, onset of frailty or increased long-term care dependency, are frequent. Prevention of infections e.g. by vaccination is therefore an important measure to ensure healthy aging and preserve quality of life. Several vaccines are specifically recommended for older adults in many countries, and in the current SARS-CoV-2 pandemic older adults were among the first target groups for vaccination due to their high risk for severe disease. This review highlights clinical data on the influenza, Streptococcus pneumoniae and herpes zoster vaccines, summarizes recent developments to improve vaccine efficacy, such as the use of adjuvants or higher antigen dose for influenza, and gives an overview of SARS-CoV-2 vaccine development for older adults. Substantial research is ongoing to further improve vaccines, e.g. by developing universal influenza and pneumococcal vaccines to overcome the limitations of the current strain-specific vaccines, and to develop novel vaccines against pathogens, which cause considerable morbidity and mortality in older adults, but for which no vaccines are currently available. In addition, we need to improve uptake of the existing vaccines and increase awareness for life-long vaccination in order to provide optimal protection for the vulnerable older age group.
Low birth rates and higher life expectancy are transforming the age pyramid in Europe towards a much older population. Between 2010 and 2020 the share of the population older than 65 years of age increased by 3 percentage points in the EU (from 17.6 to 20.6%) and is projected to further increase to more than 31% by 2100. The share of those older than 80 years is projected to have a 2.5-fold increase (5.9% to 14.6%) from now until 2100 [ 1 ]. This leads to challenges of societies, economies and health care systems on many levels. Preserving good health in old age is of utmost importance to alleviate some of these challenges, but of course also for the well-being and quality of life for every individual person. This review focusses on vaccines to prevent infectious disease in older adults. The incidence of many infections is higher in older compared to younger adults [ 2 ] and morbidity as well as mortality due to infectious diseases is increased in this age group. In addition to the immediate impact of the disease many older persons do not recover fully after an acute episode of infection and chronic co-morbidities might be exacerbated. These phenomena have been described for influenza, pneumococcal disease and herpes zoster and can lead to long-term sequelae such as onset or increase of frailty, impairments in activities of daily living and even loss of independence [ 3 , 4 , 5 ]. Prevention of infections e.g. by vaccination is therefore an important measure to ensure healthy aging and preserve quality of life.
Influenza causes approximately 15,000-70,000 deaths annually in Europe, mainly in older adults [ 6 ], and complications such as exacerbations of underlying pulmonary disease or bacterial co-infections further increase the burden of disease [ 5 , 7 ]. Vaccination against influenza is recommended in many countries for everybody, but is particularly relevant for older adults and other risk groups. Historically, influenza vaccines (TIV, trivalent influenza vaccine) contained two influenza A strains (H1N1 and H3N2) and one B strain (Yamagata or Victoria lineage). Influenza viruses are highly variable with antigenic shift (exchange of RNA segments leading to novel strains, e.g. H5N1) leading to pandemics and antigenic drift (point mutations) necessitating annual adjustments of the seasonal vaccines. Based on world-wide surveillance data WHO defines the exact composition for the annual vaccine each year. As both B lineages have co-circulated for several years, quadrivalent influenza vaccines (QIV) containing two A and two B strains have been developed and are now in widespread use [ 8 ]. Most influenza vaccines are produced by an egg-based manufacturing process, in which candidate vaccine viruses are propagated in fertilized hen´s eggs. Alternatively, influenza vaccine viruses can be grown in mammalian cell culture. Standard influenza vaccines are either split virus vaccines, which comprise disrupted viral envelopes, or subunit vaccines, for which the viral nucleocapsid is removed in further purification steps [ 9 ]. In addition, recombinant influenza vaccines use hemagglutinin proteins expressed in insect cells using a baculovirus system.
Immunogenicity
Immunogenicity of standard TIV is lower in older compared to young adults [ 10 ] and frailty and co-morbidities further decrease vaccine-induced immune responses [ 11 , 12 ]. It has also been reported that influenza-specific antibody titers decline faster in older persons leading to loss of seroprotection until the following season, or even towards the end of the same season for some influenza strains [ 13 , 14 ]. Antibodies against the viral hemagglutinin are widely used as surrogate of protection in the context of influenza vaccination [ 15 ], but might not be an ideal measure in older adults, as vaccinees with low titers may be still protected and vice versa [ 16 ]. It has been shown that memory B cells and plasmablasts are retained in older adults, despite lower antibody titers compared to young adults even after repeated vaccination. Impaired differentiation from memory B cells towards plasma cells might be responsible for this phenomenon [ 17 ]. In addition to antibodies, cell-mediated immune responses are also important to combat influenza virus infection and T cell parameters (e.g. IFN-γ and IL-10 production, Granzyme B activity) might be better predictors of clinical protection [ 18 , 19 , 20 ].
Clinical efficacy and effectiveness
In placebo-controlled trials enrolling young adults TIV is up to 70% effective against laboratory-confirmed influenza [ 21 , 22 ], but lower efficacy has been observed in older adults [ 23 , 24 ]. Meta-analysis of influenza vaccine immunogenicity, efficacy and effectiveness is difficult as different vaccine formulations are used (split vs. subunit), vaccine composition changes from year to year, clinical endpoints are variable (influenza-like illness, laboratory-confirmed influenza, hospitalization, etc.) and history of exposure (infection and prior vaccination) may vary between cohorts. Despite these uncertainties there is consensus that standard influenza vaccines are less immunogenic and efficient in older compared to younger adults and that improved vaccines for the older population are necessary.
Strategies for improvement
An obvious strategy to improve immunogenicity of vaccines might be to increase the amount of antigen per dose. A higher antigen dose should result in increased uptake and presentation by antigen-presenting cells and therefore in stronger activation of adaptive immune cells. In the US, Canada, Brazil, Australia and the UK, a trivalent high-dose influenza vaccine has been used for several years (HD-TIV). This vaccine contained 60μg hemagglutinin for each strain instead of the standard 15μg. HD-TIV was shown to induce higher anti-hemagglutinin antibody concentrations and seroprotection rates, as well as increased numbers of influenza-specific T cells in older adults compared to TIV [ 25 , 26 ]. Clinical efficacy of HD-TIV compared to standard TIV in older adults was summarized in meta-analyses, which reported a lower risk to develop laboratory-confirmed influenza (relative risk 0.76) [ 27 ] and a higher relative efficacy against pneumonia (rVE 24.3%), hospitalization for influenza (rVE 17.8%), and influenza-like illness (rVE 19.5%) [ 28 ].
An alternative strategy to improve vaccine-induced immune responses is the use of adjuvants in order to protect cohorts, which develop unsatisfactory immune responses after standard immunization (e.g. older adults). Aluminum salts have been used in human vaccines for approximately 100 years in combination with various, but not with influenza antigens. As one of the first modern adjuvants the oil-in-water emulsion MF59, which comprises squalene and the surfactants Tween 80 and Span85 was licensed as an adjuvant in seasonal influenza vaccines for older adults in 1997 (adjuvanted TIV, aTIV). It has also been approved for other age groups including children (6-24 months) and in pandemic influenza vaccines. MF59 induces proinflammatory chemokines and cytokines at the site of injection thereby recruiting innate immune cells and facilitating efficient antigen uptake, enhances differentiation of dendritic cells in the lymph nodes, and is involved in shaping the germinal center reaction [ 29 , 30 ]. Antibody responses following aTIV are slightly higher compared to TIV (summarized in [ 31 , 32 ]), and the CD4 + T cell response is elevated [ 33 ]. Interestingly, antibodies elicited by aTIV recognize drifted influenza strains more efficiently as antibodies against additional epitopes within the influenza hemagglutinin are induced [ 34 , 35 ]. A meta-analysis reported greater efficacy of aTIV in preventing laboratory-confirmed influenza (adjusted odds ratio 0.37; 95%CI: 0.14–0.96) and hospitalizations due to pneumonia/influenza (adjusted risk ratio 0.75; 95% CI: 0.57–0.98) compared to standard TIV [ 36 ].
Clinical effectiveness of HD-TIV and aTIV were recently compared directly in two studies. A retrospective cohort study, which analyzed data of persons above 65 years during the 2016/2017 and the 2017/2018 influenza seasons reported that HD-TIV provided better protection from respiratory-related hospitalizations compared to aTIV, with a pooled relative vaccine effectiveness (rVE) of 12% (95% CI, 3.3%-20%) [ 37 ]. In contrast, in a similar study effectiveness against any influenza-related medical encounter was higher for the adjuvanted trivalent vaccine (rVE 7.7%; 95%CI: 2.3%-12.85) compared to the high-dose trivalent vaccine during the 2017/2018 influenza season. Similar results were obtained for the following season [ 38 ]. The challenges in comparing different studies investigating efficacy and/or effectiveness of influenza vaccines have been mentioned above.
Both high-dose and adjuvanted influenza vaccines have recently been modified to contain four instead of three different influenza strains (HD-QIV and aQIV) and have been licensed in many countries. Recent studies confirmed higher immunogenicity of HD-QIV compared to standard QIV [ 39 ] and non-inferiority to HD-TIV [ 40 ] as well as a satisfactory safety profile. Immunogenicity and safety of aQIV have been shown to be similar to aTIV in older adults [ 41 ].
Annual vaccination against influenza is recommended for all adults in many countries, but most recommendations emphasize the particular importance for vulnerable groups, such as older adults. Several countries specifically recommend the high-dose and/or adjuvanted influenza vaccines for older adults. In previous years these guidelines were complicated by the fact that these “improved” vaccines were only trivalent, whereas the standard vaccine had already been available in a quadrivalent formulation. For the season 2021/2022 HD-QIV and aQIV will be available in many countries and are included in specific national recommendations for older adults. As examples, Germany issued its first recommendation for a specific vaccine, namely the HD-QIV, for the 2021/2022 season [ 42 ]. In the UK, aTIV or HD-TIV was recommended for older adults during the 2020/2021 season [ 43 ], whereas for the 2021/2022 season aQIV or HD-QIV should be used [ 44 ]. In contrast, the Advisory Committee on Immunization Practices in the US did not recommend a specific vaccine formulation for the older population for 2020/2021 despite the fact that adjuvanted and high-dose quadrivalent formulations were expected to be available [ 45 ], and specific recommendations for 2021/2022 were not yet available at the time of publication.
Future developments
Next-generation influenza vaccines are being developed. One approach is the use of alternative adjuvants, which might overcome the diminished responsiveness of the aged immune system. As mentioned above, MF59 is used successfully in influenza vaccines and other squalene-based adjuvants have also been tested (summarized in [ 46 ]). AS03 has been used in the pandemic influenza vaccine of 2009 and induced higher antibody concentrations and seroprotection levels in older adults compared to a whole-virus vaccine or a non-adjuvanted split vaccine [ 47 , 48 ]. In both studies the amount of antigen in the adjuvanted vaccine was substantially lower than in the comparator vaccines. Superior clinical efficacy of seasonal TIV adjuvanted with AS03 compared to standard TIV could be demonstrated for protection against influenza A, and particularly A/H3N2 infection, but not against infection with any influenza strain. In addition this study showed higher efficacy against hospital admission for pneumonia and all-cause death in descriptive estimates [ 49 ]. AF03 (squalene-based emulsion), Advax-CpG55.2 (inulin + TLR9-agonist), GLA-SE (emulsion of TLR4-agonist) and several other TLR-agonists and alternative emulsions have been tested in combination with influenza antigens and there are many more potential adjuvants, such as e.g. cytokines, T cell stimulating adjuvants, DNA-based adjuvants etc., which might be good candidates to boost vaccine-induced immune responses in older adults, but have not yet been tested in this age group. More extensive reviews on adjuvants for influenza vaccines can be found elsewhere [ 30 , 50 ]. After the rapid success of mRNA vaccines against COVID-19 (see below), several manufacturers started or intensified development of mRNA vaccines against influenza. The use of mRNA vaccines against influenza was first suggested in 2012 and it has been shown that this vaccine candidate offers protection from influenza in animal models including aged mice [ 51 ]. Compared to “traditional” influenza vaccine manufacturing in eggs or cell culture, mRNA vaccines could be produced much faster in large quantities. This is particularly relevant for influenza vaccines, as a later decision for a specific seasonal vaccine composition could improve the match between vaccine and circulating virus strains. In July 2021 Moderna started the first Phase I/II clinical trial with a seasonal influenza mRNA vaccine in humans (NCT04956575).
Influenza-specific antibodies show some degree of cross-reactivity towards related viral strains and influenza-specific T cells can recognize conserved epitopes. However, clinical data show that a mismatch between vaccine strains and circulating virus strains decreases efficacy and effectiveness of influenza vaccines. Overall influenza vaccine effectiveness is usually 40-60% in years with adequate matching of vaccine and circulating virus strains [ 52 ]. But as an example, in the 2014/2015 season vaccine effectiveness was only 19% against influenza and only 6% against H3N2 strains [ 52 ]. The circulating influenza A H3N2 strains had changed significantly (antigenic drift) after selection of the vaccine strains [ 53 ] leading to a pronounced mismatch in this year. Similar effects were observed in the seasons 2004/2005 and 2005/2006 [ 54 ]. Adjuvants can improve the production of cross-reactive antibodies which recognize drifted strains (summarized in [ 55 ]), but universal influenza vaccines would be desirable to address the immense antigenic variability of influenza viruses and to be prepared for novel, potentially pandemic influenza strains. Various approaches, such as antigens based on the conserved stem-region of hemagglutinin, chimeric hemagglutinin proteins, peptides and nucleic acid platforms, are currently tested in clinical trials. While cross-reactive or broadly reactive antibodies contribute to a broader or potentially universal protection, T cells also play a crucial role. CD4 + T cells contribute to an efficient immune response in several ways. They have direct effector functions e.g. cytokine secretion and cytolysis in the lung, are important for rapid innate responses and provide help to B cells, which ensures optimal antibody production, as well as to CD8 + T cells. The cytotoxic CD8 + T cells recognize and eliminate infected cells, thereby limiting virus spread in the body. Many of the vaccine candidates for universal influenza vaccines utilize antigens and/or technologies, which aim to elicit robust T cell responses. A comprehensive summary of these approaches has recently been published [ 56 ]. It is very likely that adjuvants and/or vaccine-delivery platforms will be essential for optimal vaccine responses, particularly in the older population [ 57 , 58 , 59 ].
Pneumococcal disease
Streptococcus pneumoniae (pneumococcus) can be classified into more than 90 distinct serotypes based on their polysaccharide capsule of which only a limited number are pathogenic [ 60 ]. Antimicrobial resistance of S. pneumoniae is an increasing problem [ 61 ]. Clinical presentation of S. pneumoniae infection can be non-invasive (otitis media, sinusitis, conjunctivitis, pneumonia) or invasive (bacteremic pneumonia, meningitis, sepsis). Incidence of invasive pneumococcal disease (IPD) as well as pneumococcal pneumonia increases with age. S. pneumoniae is the most frequently isolated pathogen causing community-acquired pneumonia (CAP) in older adults. In the US nearly 30,000 cases of invasive pneumococcal disease (IPD) and over 500,000 cases of non-bacteremic pneumococcal pneumonia were estimated to occur every year in persons older than 50 years, resulting in more than 25,000 pneumococcus-related deaths [ 62 ]. Bacterial co- or secondary infections are frequently observed in influenza patients. The exact numbers of co-infections vary greatly in different studies; a meta-analysis reported bacterial infections in 11% to 35% of influenza patients with S. pneumoniae being the most common pathogen accounting for 35% (95% CI: 14%-56%) of all bacterial co-infections [ 63 ].
Two types of vaccines are available against S. pneumoniae ; polysaccharide vaccines (PPV), which contain the purified bacterial capsule polysaccharides, and conjugated vaccines (PCV), for which the polysaccharides are conjugated to carrier proteins. The serotype coverage of the different vaccines is summarized in Table 1 .
Purified polysaccharides are T cell-independent antigens, and as such elicit a distinct immune response. Without T cell help, B cell activation and differentiation happen independently of germinal centers resulting in short-term antibody production, a lack of B cell memory and mainly IgM and IgG 2 responses [ 64 , 65 ]. Infants are not able to mount efficient immune responses against polysaccharide antigens in the first two years of life. Therefore, the PPV-23 vaccine is not suitable for young children and has only been licensed for adults despite the fact that non-invasive and invasive pneumococcal disease is highly prevalent in infants. PPV-23 has been recommended and utilized in many countries for the older population for several decades. Carrier proteins, which are chemically conjugated to the polysaccharides facilitate T cell-dependent immune responses where carrier-specific T cells provide T cell help for polysaccharide-specific B cells [ 65 ]. Class switch and avidity maturation can take place, memory B cells are generated, and the conjugate vaccines (PCV) are immunogenic in infants. PCV-7 was introduced in the late 1990s/early 2000s for young children, but was not licensed for adults. The incidence of IPD in the target age group declined substantially after introduction of this vaccine. As PCV does not only prevent disease, but also carriage of S. pneumoniae , the incidence of IPD also decreased in older adults, presumably due to reduced transmission from children to older adults [ 66 ]. A certain degree of serotype replacement was observed in all age groups in the following years leading to the development of PCV-10 and PCV-13, which contain additional serotypes and replaced PCV-7 in the childhood vaccination programs of many countries. PCV-13 was the first PCV, which was also licensed for adults and is now used in many countries for the older population. Serotype replacement can still be observed and development of PCVs containing even more serotypes is ongoing (see below).
Two different methods are used to quantify polysaccharide-specific antibodies and to determine immunogenicity of the pneumococcal vaccines. ELISA-based methods detect IgG antibodies but no other antibody classes and early ELISA methods were often unspecific. Improved protocols solved this problem, but some older studies need to be interpreted with caution [ 67 ]. Functional antibodies can be measured by opsonophagocytosis assays (OPA). In young children, these two assays show a strong correlation, but this is not the case in older adults and immunocompromised patients [ 68 ]. It has been concluded from several studies [ 69 , 70 , 71 , 72 , 73 ] that OPA-measurements are probably a more reliable correlate of protection and that they should be utilized despite the fact that they are more complex and expensive and less standardized than ELISA methods.
With age, immunogenicity of PPV-23 decreases, as shown by reduced opsonophagocytic activity, alterations in class and subclass usage as well as in somatic hypermutation [ 74 , 75 , 76 ]. Currently, PPV-23 and PCV-13 are available for older adults, but many studies comparing immune responses to PPV and PCV in this age group were already performed with PCV-7. Some studies reported higher antibody levels (ELISA and OPA) for PCV-7 [ 77 ], whereas others did not detect significant differences between antibodies elicited by PCV-7 or PPV-23, respectively [ 78 , 79 , 80 ]. This might be explained by the fact that the patient populations were heterogenous, most studies were relatively small and previous vaccination with PPV-23 impacts the response to PCV-7. Frailty further impairs antibody responses after pneumococcal vaccination [ 78 ]. More recent studies compare PPV-23 and PCV-13 and systematic meta-analyses show higher antibody levels for the majority of serotypes and non-inferiority for the others after vaccination with PCV-13 [ 81 , 82 ]. Early studies showed that a second dose of PCV-7 one year after either PPV-23 or PCV-7 might be beneficial for antibody responses and particularly for their long-term maintenance [ 77 , 79 ] and a more recent meta-analysis concluded that prior vaccination with PPV-23 did not influence the immunological response to PCV-13 [ 81 ].
Efficacy and effectiveness of pneumococcal vaccines have been mainly studied for IPD and in some studies for pneumococcal pneumonia. In an extensive meta-analysis pooled vaccine efficacy/effectiveness (VE) of PPV-23 against IPD and pneumococcal pneumonia was calculated for different types of studies and ranged from 73% against IPD in clinical trials to below 50 % in cohort studies (Table 2 ). The authors suspected a high risk of bias in the diagnosis of pneumococcal pneumonia for several studies, which they excluded from their analysis. However, these studies had been included in previous meta-analyses which failed to demonstrate a protective effect against pneumonia [ 84 , 85 , 86 , 87 ]. Clinical efficacy of PCV-13 was tested in a large Phase IV randomized, placebo-controlled trial including more than 84,000 older adults. In the per-protocol analysis PCV-13 was 45.6% (95% CI: 21.8-62.5%) effective against first episodes of community-acquired pneumonia (CAP) caused by vaccine-type strains and 75.0% (95% CI: 41.1-90.8%), against vaccine-type IPD, respectively [ 88 ]. It is still controversially discussed which pneumococcal vaccination strategy provides optimal protection against disease, as data on clinical efficacy of schedules combining PPV and PCV or repeated doses of either vaccine are lacking. Antibody responses to PCV-13 are stronger and can potentially be boostered by additional doses, but due to high vaccination rates in the pediatric population and the accompanying herd immunity effects, prevalence of the PCV-13 serotypes in older adults decreases and other serotypes might become more clinically relevant. PPV-23 covers additional serotypes, but there are concerns that PPV might induce tolerance or hyporesponsiveness upon repeated vaccination, similar to the meningococcal polysaccharide vaccine [ 89 ]. Vaccination recommendations are very heterogenous in Europe, where various countries recommend either PCV-13 or PPV-23, or PCV-13 followed by PPV-23. This combination aims to exploit the advantages of both vaccines. The sequential use of both vaccines was also recommended in the US for several years, but since 2019 only PPV-23 is generally recommended and the addition of PCV-13 should be considered for the individual patient in a shared decision process [ 90 ]. The uncertainties and inconsistencies in national recommendations might contribute to the fact that vaccination coverage for pneumococcal vaccine is still low in many countries [ 91 , 92 , 93 , 94 ].
Strategies for improvement and future developments
As mentioned above development of next-generation conjugated vaccines containing additional serotypes is ongoing. Early stage clinical trials showed that safety and immunogenicity of a 15-valent and a 20-valent conjugated vaccine (Table 1 ) were similar to that of PCV-13 in older adults [ 95 , 96 ]. PCV-15 has been tested in Phase III trials in adults over 50 years of age and younger adults with various risk factors (NCT03950622, NCT03480802, NCT03950856, NCT03615482, NCT03547167, NCT03615482) demonstrating immunogenicity when administered alone or concomitantly with influenza vaccine or followed by the 23-valent polysaccharide vaccine and has very recently been licensed in the US [ 97 ]. Phase III trials for PCV-20 are ongoing in older adults (NCT03835975, NCT04875533, NCT03760146, NCT04526574). As serotype prevalence differs between children and older adults, partially due to serotype replacement processes, it might be advisable to consider the option of including different serotypes in vaccines for the different age groups.
The use of adjuvants has been successful for various protein-based vaccines, but has not yet moved beyond early development for polysaccharide or conjugate vaccines. Alum does not improve immune responses to T-cell independent antigens or conjugates [ 98 , 99 ], and various TLR agonists failed to increase antibody responses when co-administered with pneumococcal polysaccharides [ 100 ]. Several adjuvants, such as IC31 (TLR-9 agonist + antibacterial peptide) or a combination of MPL and synthetic cord factor formulated as an oil-in water emulsion showed promising results in mouse models [ 101 , 102 ], but have not yet been tested clinically.
Universal vaccines against S. pneumoniae would be needed to fully overcome the risk of serotype replacement. A whole-cell vaccine candidate and various individual protein or peptide vaccines, most of them utilizing pneumococcal histidine triad protein D (PhtD), detoxified pneumolysin derivative (PlyD) and pneumococcal surface protein (PspA) or combinations of those are developed. Many of these vaccine candidates combine the antigens with different adjuvants. Several of these vaccine candidates show promising immunogenicity and safety profiles in early clinical studies and even more are still in pre-clinical development [ 103 , 104 , 105 , 106 , 107 , 108 , 109 , 110 , 111 ].
- Herpes zoster
Primary infection with varicella-zoster virus (VZV) usually occurs in childhood and manifests as chickenpox (varicella). Life-long viral latency is established in sensory ganglia, and reactivation of VZV, which can occur throughout life, is usually controlled by T cell responses (cell-mediated immunity, CMI) and therefore asymptomatic. In situations with reduced CMI, e.g. under immunosuppression or with increasing age, reactivations can manifest as herpes zoster (HZ) if the virus spreads through the sensory nerve to the corresponding dermatome. This results in a typically unilateral, frequently painful, segmented skin rash. A substantial increase of the HZ incidence with age (2/1,000 person-years at age 50; 6–8/1,000 person-years at 60; 8–12/1,000 person-years at age 80) was reported in a systematic review, which included 130 studies from various countries [ 112 ].
Pain occurring or persisting more than 3 months after onset of the rash is referred to as post-herpetic neuralgia (PHN), and is a frequent complication of HZ. PHN is often associated with severe pain, which is very difficult to manage therapeutically and can last for several months resulting in considerable impact on activities of daily living and quality of life [ 113 , 114 ]. The incidence of PHN also increases with age from 18% in HZ patients older than 50 years to 33% in HZ patients older than 80 years [ 115 ]. HZ and PHN are prominent examples how an acute episode of infection can lead to long-term sequelae including loss of independence and institutionalization. Vaccination against HZ aims to restore the VZV-specific immune response, which was generated during the primary infection, in order to prevent the clinical consequences of viral reactivation.
A live-attenuated vaccine based on the Oka Merck virus strain is available to prevent primary infection with VZV in children, and the same strain (14-fold higher dose) was also used in older adults to prevent HZ. As a live-attenuated vaccine, it is not suitable for immunocompromised patients, who are at high risk for HZ, but it has a favorable reactogenicity and safety profile in immunocompetent persons including older adults. A second-generation vaccine against HZ contains the viral envelope glycoprotein E (gE) and the adjuvant system AS01B, which consists of 3-O-desacyl-4´-monophosphoryl lipid A (MPL, a derivative of lipopolysaccharide) and QS-21, a saponin found in the bark of the tree Quillaja Saponaria, formulated in liposomes, which act as antigen delivery systems. This combination enables uptake of the antigen via endocytosis, activates innate immune cells via the TLR-4 pathway and targets subcapsular macrophages in the lymph node [ 116 , 117 , 118 , 119 , 120 ], leading to efficient activation of adaptive immune responses [ 121 ]. The adjuvanted vaccine was licensed and is now used in Europe, Canada, the US, Japan and many other countries.
Both vaccines induce antibody and cellular immune responses, but T cell mediated immunity (CMI) is considered to be essential for protection against herpes zoster, whereas only a minor role is attributed to antibodies. Immunogenicity was investigated in sub-cohorts of the pivotal Phase III trials for both vaccines. It has been shown that baseline VZV-specific T cell responses decline with age and are lower for persons ≥70 compared to those 60-69 years of age, whereas baseline antibody levels are independent of age and did not correlate with CMI [ 122 ]. Upon vaccination with the live-attenuated vaccine VZV-specific T cell responses increase and although the magnitude of these responses decreases over time, particularly within the first year, CMI remains above baseline levels for the observation period of three years. Similar observations were made for VZV-specific antibodies. VZV-CMI is significantly lower in subjects ≥70 compared to the 60-69 years-old cohort, but no age-related differences in antibody responses were observed. Despite an inverse correlation of VZV-CMI with the likelihood of developing HZ this study was not able to identify a surrogate marker or threshold level of protection [ 122 ]. Upon vaccination with two doses of the recombinant adjuvanted vaccine VZV-CMI (measured by gE-specific CD4 + T cells producing at least two cytokines) increases in more than 90% of the recipients and remains above the CMI-response threshold in 57% three years later. Specific CD4 + T cells persist substantially above pre-vaccination values even 9 years after vaccination [ 123 ]. A slightly lower proportion of vaccine recipients ≥70 remains above this threshold compared to younger participants confirming previous data on a moderate age-associated decline of T cell, but not antibody responses [ 124 , 125 , 126 ]. CD8 + T cell responses were rarely detected in this study. Antibody levels also increase substantially and remain over baseline for at least three years. Only minimal differences were observed between age groups at any time point. In contrast to the study with the live-attenuated vaccine, a moderate positive correlation between humoral and CMI responses was observed after vaccination with the recombinant vaccine [ 127 ]. Direct comparisons of T cell and antibody responses induced by the two vaccines are complicated by the fact that the live-attenuated vaccine contains a multitude of viral antigens whereas the recombinant vaccine includes only the glycoprotein gE. gE-specific CD4 + T cell responses are substantially higher following vaccination with the recombinant vaccine, but even total VZV-specific T cell responses were not superior following vaccination with the live-attenuated vaccine. In addition, the recombinant vaccine induced preferentially memory, but less effector T cells. The authors of this study conclude that gE-specific memory Th1-responses are relevant for protection and that high peak memory T cell responses are necessary for long-term persistence [ 128 ]. Antibody responses measured by ELISA are also higher after vaccination with the recombinant vaccine. Interestingly, the avidity of gE-specific antibodies at baseline (i.e. before vaccination) is much lower compared to antibodies specific for all VZV-glycoproteins and vaccination with the recombinant, but not the live-attenuated vaccines substantially improves avidity of the gE-specific antibodies. In addition, induction of neutralizing antibodies is superior after vaccination with the recombinant vaccine. In summary, the quality of gE-specific antibodies seems to be higher after vaccination with the recombinant vaccine. The exact role of these highly functional antibodies for protection remains to be elucidated [ 129 ].
Vaccine efficacy in persons older than 60 years was 51% against HZ and 67% against PHN in the pivotal Phase III randomized, double-blinded, placebo-controlled trial for the live-attenuated vaccine [ 130 ]. However, as mentioned above reduced T cell responses were observed in the oldest participants, which was also reflected in lower efficacy (60-69y: 64%; 70-79y: 41%; ≥80y: below 20%) in the pivotal trial and in an additional study, which showed higher efficacy of 70% in a younger cohort aged 50-59 years [ 122 , 131 ]. The protective effect of the vaccine waned over time and was lost approximately 10 years after vaccination [ 132 ], but re-vaccination after 10 years results in a booster effect and restores immune responses [ 133 ].
The two pivotal phase III randomized, placebo-controlled clinical trials for the recombinant, adjuvanted vaccine included more than 30,000 participants older than 50 or 70 years, respectively [ 134 , 135 ]. No major safety concerns were identified after two doses of vaccine administered 8 weeks apart. The majority of adverse effects were temporary reactions at the site of infection and systemic symptoms, such as headache, myalgia or fatigue were relatively frequent, but mild. Clinical efficacy against HZ was 97.2% (95% CI: 93.7-99.0) in persons over 50 and 89.8% (95% CI: 86.8-94.5) in persons over 70 years of age, respectively in the two phase III trials. Combined analysis of all participants did not reveal statistically significant differences between age groups 70-79 and ≥80 years [ 134 , 135 ]. Despite a slight decline, efficacy remained above 85% for the first four years after vaccination and did not decrease further until 7 years post-vaccination [ 136 ]. Post-herpetic neuralgia was very rare in the vaccinated study cohort, with no cases in participants younger than 70 years and a vaccine efficacy of 88.8% (95% CI: 68.7-97.1) in persons older than 70 [ 134 ].
Frailty status was determined for more than 90% of the participants of the two studies, and as expected, prevalence of frailty increased with age. Vaccine efficacy against HZ was above 90% also in the frail and pre-frail sub-cohorts [ 137 ]. Vaccine effectiveness was lower in an observational post-licensure study, namely 70.1% (95%CI: 68.6-71.5), but did also not decline with age (65-79y vs. ≥80y). This study showed that the second dose is required for optimal protection, but that a delay to administer the second dose (>180 days after dose 1) does not impair protection [ 138 ].
In contrast to the live-attenuated vaccine, the recombinant vaccine is suitable for immunocompromised patients. Safety and immunogenicity have been demonstrated in patients after renal transplantation [ 139 ], in HIV-positive patients [ 140 ] and in patients receiving chemotherapy or immunosuppressive treatment for hematologic malignancies [ 141 ].
A clear limitation of the live-attenuated vaccine is that its use is contraindicated for patients under immunosuppression. An inactivated vaccine, which used a heat-inactivated formulation of the Oka Merck virus strain demonstrated 63.8% (95%CI: 48.4-74.6) and 83.7% (95%CI: 44.6-95.2) efficacy against HZ or PHN, respectively in a Phase III trial in hematopoietic stem-cell transplant recipients [ 142 ]. However, four doses are required, and with the licensure of the recombinant, adjuvanted vaccine (see above), which is also suitable for immunocompromised patients it seems unclear how this vaccine will be used. There is also ongoing development of an alternative subunit adjuvanted vaccine (NCT03820414).
In the current SARS-CoV-2 pandemic older adults, persons with underlying co-morbidities and obese individuals have the highest risk for severe disease and death from COVID-19 [ 143 , 144 , 145 ]. Reports of long-lasting health sequelae after recovery from COVID-19 (“long COVID”) are accumulating [ 146 , 147 ], but this complication seems to be more frequent in younger patients. Efficient vaccines are essential to control the pandemic and older adults are an important target group for vaccination against SARS-CoV-2. Most national vaccination programs prioritized old adults and this age group was among the first recipients of SARS-CoV-2 vaccines. Several vaccines against SARS-CoV-2 have been licensed in different countries, clinical trials with additional vaccine candidates are ongoing and a plethora of vaccine candidates are in pre-clinical development [ 148 ]. This summary focusses on the SARS-CoV-2 vaccines currently licensed in Europe, namely the mRNA-based vaccines of BioNTech/Pfizer (Comirnaty) and Moderna (Spikevax) and the adenoviral vectors of AstraZeneca (chimpanzee adenovirus ChAdOx1; Vaxzevria) and Johnson&Johnson/Janssen (human Adenovirus-26; COVID-19 Vaccine Janssen) and highlights their safety, immunogenicity, efficacy and effectiveness in older adults. With the exception of Vaxzevria, they are also licensed for emergency use in the US. All four vaccines deliver genetic information for the SARS-CoV-2 spike protein, which is then produced by cells of the vaccinee. Comirnaty, Spikevax and Vaxzevria are administered in a two-dose regimen. COVID-19 vaccine Janssen is a single-dose vaccine. A complete overview of SARS-CoV-2 vaccine development is beyond the scope of this article and can be found elsewhere [ 149 ].
First-in-human clinical trials are usually performed in young, healthy adults, but for SARS-CoV-2 vaccines clinical development moved quickly towards the inclusion of older adults. As an example, the phase I dose escalation trial for Spikevax was extended from the original cohort (18-55 years) to also include participants >55 and >70 years [ 150 ] and Comirnaty was also tested early in the age groups 18-55 and 65-85 years [ 151 ]. Both studies demonstrated similar immunogenicity for the different age groups. The phase I/IIa study for COVID-19 vaccine Janssen included adults aged 18-55 or more than 65 years, respectively. Antibody and CD4 + T cell responses after one dose of vaccine were slightly lower in the older age group. The age-associated decrease in immunogenicity was more pronounced for CD8 + T cell responses, with cytokine-producing T cells detectable in 51% of the younger and 36% of the older cohort, respectively [ 152 ]. Phase II and III studies of all vaccines also investigated immunogenicity and confirmed these results. Antibody levels and neutralizing titers, as well as T cell responses after vaccination with Vaxzevria were similar in the age groups 18-55y, 56-69y, and ≥70y [ 153 ]. Further immunogenicity trials including older adults are still ongoing for the different vaccines, and now also include the newly emerging virus variants. Neutralizing antibodies against the original virus after the first dose of BioNTech/Pfizer mRNA vaccine are substantially lower in persons older than 80 years compared to younger age-groups, and this effect is even more pronounced for virus variants. However, the age-associated differences were less prominent after completion of the two-dose vaccination series. In addition, differences in B cell memory, somatic hypermutation and T cell responses were observed comparing persons younger or older than 80 years [ 154 ].
Safety of all vaccines has been investigated extensively. This summary highlights age-related aspects and therefore mainly relies on the safety data from later stage trails, which included more older participants. The safety profile of the two mRNA vaccines Comirnaty and Spikevax are similar, with local pain at the site of injection being more frequent than redness and swelling. Fatigue, headache, muscle pain and chills are the most frequent systemic events [ 155 , 156 ]. The reactogenicity profiles of the vector vaccines Vaxzevria and COVID-19 vaccine Janssen are similar, with pain and tenderness reported as the most common local and fatigue and headache as the most common systemic reactions [ 152 , 153 , 157 ]. Local and systemic reactogenicity was higher after the second than after the first dose of mRNA vaccines, but higher after the first dose of Vaxzevria, compared to the second dose of this vaccine. For all vaccines, reactogenicity was lower in older compared to younger adults. Severe adverse events were rare for all vaccines and mostly not vaccine related. After introduction of the vaccines and use in a large number of persons very rare severe adverse events were observed including allergic reactions, mainly against the mRNA vaccines [ 158 , 159 ] and thrombosis with thrombocytopenia syndrome (TTS) after vaccination with the adenoviral vector vaccines [ 160 ]. Rare cases of myocarditis have been reported in adolescents and young adults (<30y) after vaccination with mRNA vaccines [ 161 ]. These rare complications are more relevant for younger adults and will therefore not be discussed in detail.
Phase III clinical trials to determine vaccine efficacy included older adults, but particularly for the oldest age groups the number of participants was usually not sufficient for statistical analysis. For the BioNTech/Pfizer vaccine, overall efficacy against symptomatic SARS-CoV-2 infection was 95.0 % (95%CI: 90.0-97.9) after 2 doses with a 3-week interval. Vaccine efficacy did not differ in the age groups 16-55y, >55y, and ≥65y. However, analysis of the oldest age group (≥75y) was not statistically significant with 5 cases of COVID-19 in the placebo and zero cases in the vaccinated cohort, respectively. In this study more than 40% of the participants were older than 55 years, but less than 5% were over 70 years old [ 156 ]. Results were similar for the second mRNA vaccine, Spikevax. Approximately 25% of the participants in this pivotal phase III study were older than 65 years. The vaccine interval was 4 weeks between the two doses and overall efficacy against symptomatic SARS-CoV-2 infection was 94.1% (95%CI: 89.3-96.8) after the second dose. For the different age groups efficacy was 95.6% (95%CI: 90.6-97.9) and 86.4% (95% CI: 61.4-95.2) for participants younger or older than 65 years, respectively [ 155 ]. Additional analyses showed an efficacy of 82.4% (95%CI: 46.9-93.9) for persons aged 65-75y, and again no statistically valid results for the subgroup older than 75 years of age, as no COVID-19 cases occurred in the vaccinated group versus 7 cases in the placebo group [ 162 ]. Clinical trial results for Vaxzevria were reported as a summary of several studies with differences in the age of participants, dosing of the vaccine and time intervals between the two doses. After two full-dose vaccinations, clinical efficacy against symptomatic SARS-CoV-2 infection was 62.1% (95%CI: 41.0-75.7). Only 12% of the participants were older than 55 years and no age-stratified sub-analysis was provided [ 163 ]. As a result, some countries recommended this vaccine only for younger adults at the beginning of their vaccination campaigns. In March 2021, press releases announced 79.9% efficacy against symptomatic disease in persons older than 65 years for an ongoing Phase III trial in the US, but the full data are not published yet (NCT04516746; [ 164 ]). In the pivotal trial for the single-dose COVID-19 vaccine Janssen clinical efficacy against moderate to severe COVID-19 disease was reported to be 66.1% (95% CI: 55.0-74.8). There were no differences between the age groups (younger or older than 60 years) with 33% of the participants falling into the older age group [ 152 ]. Vaccine efficacy against asymptomatic infection is of particular interest from a public health perspective, because potential transmission of the virus from vaccinated, asymptomatically infected individuals might play an important role in overall spread of the virus. In the phase III trials, different strategies were implemented to detect asymptomatic infections. A sub-cohort of the participants in the Vaxzevria studies was monitored for asymptomatic infection by weekly self-swabs and PCR testing, but efficacy was not statistically significant after receiving two full-dose vaccinations [ 163 ]. In contrast, the investigators of the COVID-19 vaccine Janssen determined antibodies against the viral N protein 71 days after the vaccination. As this antigen is not present in the vaccine, this assay identifies persons who had been infected. Vaccine efficacy against asymptomatic infection was 65.5% (95%CI: 39.9-81.1) in this preliminary analysis [ 152 ]. The phase III trials with the two mRNA vaccines announced to provide similar seroconversion data after a longer observation period.
Due to the limited supply of vaccines at the beginning of national vaccination campaigns specific target groups including health care workers and older adults were prioritized and were the first to receive the vaccine. Effectiveness in “real life” has been and is still monitored in many countries. Asymptomatic infections were mainly monitored in health care workers as they are routinely tested for active infection irrespective of vaccination. High effectiveness of the mRNA vaccines was demonstrated already after the first dose and further increased after full vaccination [ 165 , 166 ]. However, these studies did not provide data for older adults. Nation-wide efficacy data was first available from Israel, where the vaccination campaign with Comirnaty was rolled out extraordinarily fast after licensure. Vaccine effectiveness was reported against asymptomatic and symptomatic SARS-CoV-2 infection, as well as for COVID-19 related hospitalization and death for several age groups (16-44y; 45-64y; ≥65y). The lowest effectiveness was 85.9% (95%CI: 80.2-89.9) for asymptomatic infection in the oldest age group, all other outcomes were higher, most of them above 95% [ 167 ]. The oldest age group was further stratified, but no differences were observed between the age groups ≥65y, ≥75y, and ≥85y. These findings demonstrated excellent effectiveness against infection and disease in all age groups, including the very old. A Danish pre-print reported a vaccine effectiveness against PCR-confirmed SARS-CoV-2 infection of 64% (95%CI: 14-84) in long-term care facility residents (median age 84y) and 90% (95%CI: 82-95) in health care workers (median age 48y) more than 7 days after the second dose of Comirnaty. It has to be pointed out, that this study investigated PCR-confirmed infections, not symptomatic disease [ 168 ]. A study in Spanish long-term care facility residents provided vaccine effectiveness estimates of 71% (95% CI: 56–82%), 88% (95% CI: 75–95%), and 97% (95% CI: 92-99%), against SARS-CoV-2 infections (symptomatic and asymptomatic), COVID-19 hospitalizations and deaths, respectively. In this study both mRNA vaccines were included [ 169 ]. Additional effectiveness studies were performed in other countries and also with the adenovector vaccines, mostly confirming these results. A comprehensive review of vaccine efficacy and effectiveness can be found elsewhere [ 170 ].
Many factors are relevant for optimal protection. There is evidence that longer time-intervals between the two vaccine doses are beneficial [ 171 , 172 ] and that heterologous prime-boost schedules might induce superior immune responses [ 173 ], but most of these studies are too small to determine clinical efficacy of the different schedules. Duration of protection and as a consequence the need and optimal timing for additional vaccines doses are currently a major focus of interest. First reports are emerging that antibody and T cell responses persist for several months after vaccination [ 174 , 175 ], but it is unclear how long clinical protection lasts. It is impossible to study this question in the context of the placebo-controlled clinical trials as in the meantime vaccination was offered to the participants who originally received placebo. In July 2021 Israel reported that they observed only 64% vaccine effectiveness against infection and symptomatic infection in the previous weeks (compared to>90% in the early reports; see above), while effectiveness against serious illness and hospitalization was still 93% [ 176 ]. However, so far no scientific publications are available confirming these data. Israel decided to offer a third vaccine dose to persons older than 60 years, who received their second dose at least 5 months earlier, extending this recommendation soon thereafter to everybody older than 50, and then 40 years. Other countries, e.g. Germany also plan to administer third doses to high-risk individuals, e.g. older adults starting in autumn 2021, while US authorities do not support a third dose for immunocompetent individuals at the moment, but prepare for the possibility that the need arises. At the same time, WHO calls for halting administration of third doses at a time when a large proportion of the world´s population have not even received a first dose. This discussion is further complicated by the fact that different virus variants arose over time and have to be taken into account. At the time of the first clinical trials the “original” virus was gradually replaced by the alpha variant (B.1.117) and at the moment almost all cases e.g. in Europe and the US are caused by the delta variant (B1.617.2). Vaccine effectiveness differs for the variants, particularly after the first dose [ 177 ], and therefore direct comparisons of effectiveness at different time points after vaccination are difficult. It is important to monitor long-term protection specifically in high-risk populations, such as older adults, to make evidence-based decisions. Modified vaccines, which incorporate the crucial changes in the viral genome are being developed.
In future years, SARS-CoV-2 vaccines might become part of national vaccination schedules for everybody, or at least for risk groups. First studies are ongoing investigating co-administration of SARS-CoV-2 vaccines with other vaccines, such as influenza or pneumococcal vaccines (NCT04848467, NCT04790851), and development of combination vaccines (e.g. SARS-CoV-2 and influenza) has started [ 178 ].
Vaccines for all adults
Many countries recommend regular booster vaccinations against tetanus and diphtheria, sometimes in combination with acellular pertussis and/or inactivated polio for all adults. Other vaccines might be relevant in some countries, e.g. against tick-borne encephalitis in endemic areas. Most recommendations for these vaccines do not mention older adults in particular, but some countries e.g. in Europe Austria, Liechtenstein, France and Portugal advocate shortened booster intervals for older adults. Tetanus- and even more so diphtheria-specific antibody concentrations are frequently below the levels considered to be protective for adults, and are even lower in older age groups [ 179 , 180 , 181 , 182 , 183 ]. In one of our studies in Austria, booster shots with a combined vaccine containing diphtheria toxoid did not provide long-term protection in almost half of the older participants [ 180 , 184 ]. Tetanus- and diphtheria- specific antibody levels vary greatly in different European countries. In general, protection against tetanus is adequate in most countries, whereas antibodies against diphtheria are below the protective level for a substantial fraction of the population in some countries, and are decreasing with age [ 185 ]. A detailed overview on vaccination against tetanus and diphtheria can be found elsewhere [ 186 ]. Antibody responses after booster vaccination against tick-borne encephalitis are also lower in old compared to young adults and decline over time [ 187 , 188 ]. Pertussis infection can be severe in older adults and increased numbers of cases have been observed in the last years in this age group [ 189 , 190 , 191 ]. To prevent not only severe cases in adults but also transmission to newborns, who are too young to be vaccinated, vaccination against pertussis is important for adults. Regular booster doses of Tdap (tetanus, diphtheria, acellular pertussis) vaccine are well tolerated and immunogenic in young and older adults, but antibody concentrations are lower in the older age groups [ 179 , 192 ]. However, only few countries recommend regular booster immunization with combination vaccines containing the pertussis component and some recommend one booster dose during adulthood.
As mobility, financial resources and health of older adults have improved over the last decades, long-distance travel and as a consequence the need for travel vaccines have become increasingly common for this age group. Despite the fact that some tropical diseases, e.g. Japanese encephalitis and typhoid fever are more frequent and severe in older adults [ 193 , 194 ], only limited data are available on immunogenicity and efficacy of travel vaccines in older age groups. Immunization guidelines rely primarily on studies with young adults. A detailed review of travel vaccines for older adults is beyond the scope of this article and can be found elsewhere [ 195 , 196 ]. The loss of naïve T and B cells is a hallmark of immunosenescence, and an impaired generation of memory responses has been reported in aged animals [ 197 , 198 ]. This suggests that responses to neo-antigens, such as travel vaccines, might be particularly impaired in old age. Antibody responses to Hepatitis A and B vaccination are already lower in middle-aged adults and non-responders to Hepatitis B vaccine are more frequent in older age groups [ 199 , 200 , 201 , 202 ]. It has to be emphasized that vaccination against Hepatitis B is relevant for older adults not only as a travel vaccine, but also in other settings, such as for hemodialysis patients and household contacts of infected patients. Immunogenicity and efficacy of the live-attenuated yellow fever vaccine is high even in older adults, but the risk for rare severe adverse events increases with age. Yellow-fever vaccine-associated viscerotropic disease mimics viral infection and has a mortality of up to 60% [ 203 ]. A yellow fever vaccine with an improved safety profile in the older population would be desirable.
Conclusions and Outlook
Older adults are at increased risk for severe disease caused by various pathogens and this is particularly evident in the current SARS-CoV-2 pandemic. The last 18 months have also shown that novel vaccines can successfully be developed in a short period of time. Clinical efficacy of COVID-19 vaccines is high, even in older adults. Nevertheless, it seems that immunity and potentially protection wanes faster in older age groups and that additional doses will be required earlier in these cohorts highlighting the importance of including specific age- and risk-groups in clinical and observational studies. Several vaccines against other pathogens, such as influenza, S. pneumoniae and herpes zoster are available for older adults, and vaccines that are recommended for all adults (e.g. diphtheria, tetanus, pertussis) are also relevant in old age. Modifications of the vaccines, such as higher antigen dose or adjuvants for influenza vaccines, and optimization of vaccination schedules are strategies to improve vaccine effectiveness for older adults. However, vaccination coverage is still unsatisfactory for many of these vaccines and systematic data on vaccine uptake are not collected in all countries. Barriers to vaccine uptake are manifold and include lack of access, which can be caused by financial or other constraints, and personal decisions against vaccination based on missing information or misinformation regarding risk of disease and benefit of vaccination. To overcome these issues documentation of vaccine uptake in conjunction with data such as age and other risk factors is crucial in order to identify gaps in coverage and develop strategies to specifically target the relevant cohorts. Vaccination documentation is important also on a per-person scale in order to deliver vaccines at the right time and to enable reminder systems.
There are still many pathogens, which cause high morbidity and mortality in the older population, for which no vaccines are available, but would be highly desirable. The risk for nosocomial infections is high for older persons due to an increased frequency of invasive procedures, hospitalization or long-term care, and antibiotic resistance of bacterial pathogens is an increasing problem in these settings. Staphylococcus aureus and Escherichia coli , which are responsible for infections of catheters, prostheses, or surgical wounds and Clostridium difficile , which is the most common cause of nosocomial diarrhea are among the most relevant bacterial nosocomial pathogens [ 204 ]. Several potential vaccines have been clinically tested in different settings, but did not fulfil expectations, and more recent vaccine candidates are still in pre-clinical development. Vaccines against other pathogens, such as Klebsiella pneumoniae , Pseudomonas aeruginosa , Acinetobacter ssp . , and Candida spp. could have a substantial impact. Norovirus is the most relevant viral nosocomial infection and outbreaks in hospitals and long-term care facilities are frequent. The disease is characterized by vomiting and diarrhea and can be severe in older adults. In 2016 the World Health Organization stated that the development of a norovirus vaccine should be considered an absolute priority, and vaccine development is ongoing [ 205 ]. A detailed summary on vaccines for nosocomial infections of older adults has recently been published [ 206 ]. Respiratory syncytial virus (RSV) causes severe respiratory infections in infants, but older, particularly frail persons are also at high risk for severe disease. It is estimated that 18,000 hospitalizations and 8,400 deaths per year are caused by RSV in the UK, and most of these cases occur in the older population [ 207 ]. RSV vaccine development was impeded by the fact that the first RSV vaccine in the 1960s was associated with a risk for antibody-mediated disease enhancement in infants. Several novel vaccine candidates have been in clinical trials over the last years, but failed to demonstrate clinical efficacy despite encouraging safety and immunogenicity data [ 208 ]. There are many more vaccine candidates against a plethora of pathogens in various stages of development, but a complete overview is beyond the scope of this review.
Optimization of existing vaccines and vaccination strategies as well as development of novel vaccines for “old” (e.g. universal influenza or pneumococcal vaccines) and “new” pathogens have the potential to substantially improve health and quality of life in older adults. A detailed knowledge about age-associated changes of the immune system is essential in order to rationally design vaccines which hopefully overcome these limitations, and it is of utmost importance to consider age-related aspects already early in the vaccine development process (Table 3 ).
Availability of data and materials
Not applicable
Eurostat. Population structure and aging. (2021) Available at: https://ec.europa.eu/eurostat/statistics-explained/index.php?title=Population_structure_and_ageing . Accessed 18 July 2021.
Gavazzi G, Krause K. Ageing and infection. Lancet. 2002;2:659–66. https://doi.org/10.1016/S1473-3099(02)00437-1 .
Article Google Scholar
Janssens JP. Pneumonia in the elderly (geriatric) population. Curr Opin Pulm Med. 2005;11:226–30.
PubMed Google Scholar
Herpes Zoster and Functional Decline Consortium. Functional decline and herpes zoster in older people: an interplay of multiple factors. Aging Clin Exp Res. 2015;27:757–65. https://doi.org/10.1007/s40520-015-0450-0 .
Macias AE, McElhaney JE, Chaves SS, Nealon J, Nunes MC, Samson SI, et al. The disease burden of influenza beyond respiratory illness. Vaccine. 2021;39:A6–A14. https://doi.org/10.1016/j.vaccine.2020.09.048 .
Article CAS PubMed Google Scholar
European Centre for Disease Prevention and Control. Factsheet about seasonal influenza. (2021)
Google Scholar
Dugan HL, Henry C, Wilson PC. Aging and influenza vaccine-induced immunity. Cell Immunol. 2020;348:103998. https://doi.org/10.1016/j.cellimm.2019.103998 .
Tisa V, Barberis I, Faccio V, Paganino C, Trucchi C, Martini M, et al. Quadrivalent influenza vaccine: a new opportunity to reduce the influenza burden. J Prev Med Hyg. 2016;57:E28–33.
CAS PubMed PubMed Central Google Scholar
Kon TC, Onu A, Berbecila L, Lupulescu E, Ghiorgisor A, Kersten GF, et al. Influenza vaccine manufacturing: Effect of inactivation, splitting and site of manufacturing. Comparison of influenza vaccine production processes. PLoS One. 2016;11:e0150700. https://doi.org/10.1371/journal.pone.0150700 .
Goodwin K, Viboud C, Simonsen L. Antibody response to influenza vaccination in the elderly: A quantitative review. Vaccine. 2006;24:1159–69. https://doi.org/10.1016/j.vaccine.2005.08.105 .
Myśliwska J, Trzonkowski P, Szmit E, Brydak LB, Machała M, Myśliwski A. Immunomodulating effect of influenza vaccination in the elderly differing in health status. Exp Gerontol. 2004;39:1447–58. https://doi.org/10.1016/j.exger.2004.08.005 .
Article PubMed Google Scholar
Yao X, Hamilton RG, Weng NP, Xue QL, Bream JH, Li H, et al. Frailty is associated with impairment of vaccine-induced antibody response and increase in post-vaccination influenza infection in community-dwelling older adults. Vaccine. 2011;29:5015–21. https://doi.org/10.1016/j.vaccine.2011.04.077 .
Article PubMed PubMed Central Google Scholar
Carlock MA, Ingram JG, Clutter EF, Cecil NC, Ramgopal M, Zimmerman RK, et al. Impact of age and pre-existing immunity on the induction of human antibody responses against influenza B viruses. Hum Vaccines Immunother. 2019;15:2030–43. https://doi.org/10.1080/21645515.2019.1642056 .
Kissling E, Nunes B, Robertson C, Valenciano M, Reuss A, Larrauri A, et al. I-MOVE multicentre case–control study 2010/11 to 2014/15: Is there within-season waning of influenza type/subtype vaccine effectiveness with increasing time since vaccination? Eurosurveillance. 2016;21:30201. https://doi.org/10.2807/1560-7917.ES.2016.21.16.30201 .
McElhaney JE, Andrew MK, McNeil SA. Estimating influenza vaccine effectiveness: Evolution of methods to better understand effects of confounding in older adults. Vaccine. 2017;35:6269–74. https://doi.org/10.1016/j.vaccine.2017.09.084 .
Ward BJ, Pillet S, Charland N, Trepanier S, Couillard J, Landry N. The establishment of surrogates and correlates of protection: Useful tools for the licensure of effective influenza vaccines? Hum Vaccines Immunother. 2018;14:647–56. https://doi.org/10.1080/21645515.2017.1413518 .
Frasca D, Diaz A, Romero M, Blomberg BB. The generation of memory B cells is maintained, but the antibody response is not, in the elderly after repeated influenza immunizations. Vaccine. 2016;34:2834–40. https://doi.org/10.1016/j.vaccine.2016.04.023 .
Article CAS PubMed PubMed Central Google Scholar
Shahid Z, Kleppinger A, Gentleman B, Falsey AR, McElhaney JE. Clinical and immunologic predictors of influenza illness among vaccinated older adults. Vaccine. 2010;28:6145–51. https://doi.org/10.1016/j.vaccine.2010.07.036 .
Merani S, Kuchel GA, Kleppinger A, McElhaney JE. Influenza vaccine-mediated protection in older adults: Impact of influenza infection, cytomegalovirus serostatus and vaccine dosage. Exp Gerontol. 2018;107:116–25. https://doi.org/10.1016/j.exger.2017.09.015 .
McElhaney JE, Verschoor CP, Andrew MK, Haynes L, Kuchel GA, Pawelec G. The immune response to influenza in older humans: Beyond immune senescence. Immun Ageing. 2020;17:1–10. https://doi.org/10.1186/s12979-020-00181-1 .
Demicheli V, Jefferson T, Ferroni E, Rivetti A, Di Pietrantonj C. Vaccines for preventing influenza in healthy adults. Cochrane Database Syst Rev. 2018;2:CD001269. https://doi.org/10.1002/14651858.CD001269.pub6 .
Beran J, Vesikari T, Wertzova V, Karvonen A, Honegr K, Lindblad N, et al. Efficacy of Inactivated Split-Virus Influenza Vaccine against Culture-Confirmed Influenza in Healthy Adults: A Prospective, Randomized, Placebo-Controlled Trial. J Infect Dis. 2009;200:1861–9. https://doi.org/10.1086/648406 .
Govaert TME, Thijs CTMCN, Masurel N, Sprenger MJW, Dinant GJ, Knottnerus JA. The Efficacy of Influenza Vaccination in Elderly Individuals A Randomized Double-blind Placebo-Controlled Trial From the Departments of General Practice. JAMA. 1994;272:1661–5 Available at: http://jama.jamanetwork.com/ .
Kwong JC, Campitelli MA, Gubbay JB, Peci A, Winter AL, Olsha R, et al. Vaccine effectiveness against laboratory-confirmed influenza hospitalizations among elderly adults during the 2010-2011 season. Clin Infect Dis. 2013;57:820–7. https://doi.org/10.1093/cid/cit404 .
DiazGranados CA, Dunning AJ, Kimmel M, Kirby D, Treanor J, Collins A, et al. Efficacy of high-dose versus standard-dose influenza vaccine in older adults. N Engl J Med. 2014;371:635–45. https://doi.org/10.1056/NEJMoa1315727 .
Cowling BJ, Perera RAPM, Valkenburg SA, Leung NHL, Iuliano AD, Tam YH, et al. Comparative Immunogenicity of Several Enhanced Influenza Vaccine Options for Older Adults: A Randomized, Controlled Trial. Clin Infect Dis. 2019. https://doi.org/10.1093/cid/ciz1034 .
Wilkinson K, Wei Y, Szwajcer A, Rabbani R, Zarychanski R, Abou-Setta AM, et al. Efficacy and safety of high-dose influenza vaccine in elderly adults: A systematic review and meta-analysis. Vaccine. 2017;35:2775–80. https://doi.org/10.1016/j.vaccine.2017.03.092 .
Lee JKH, Lam GKL, Shin T, Kim J, Krishnan A, Greenberg DP, et al. Efficacy and effectiveness of high-dose versus standard-dose influenza vaccination for older adults: a systematic review and meta-analysis. Expert Rev Vaccines. 2018;17:435–43. https://doi.org/10.1080/14760584.2018.1471989 .
Lofano G, Mancini F, Salvatore G, Cantisani R, Monaci E, Carrisi C, et al. Oil-in-Water Emulsion MF59 Increases Germinal Center B Cell Differentiation and Persistence in Response to Vaccination. J Immunol. 2015;195:1617–27. https://doi.org/10.4049/jimmunol.1402604 .
Weinberger B. Adjuvant strategies to improve vaccination of the elderly population. Curr Opin Pharmacol. 2018;41:34–41. https://doi.org/10.1016/j.coph.2018.03.014 .
Camilloni B, Basileo M, Valente S, Nunzi E, Iorio AM. Immunogenicity of intramuscular MF59- Adjuvanted and intradermal administered influenza enhanced vaccines in subjects aged over 60: A literature review. Hum Vaccines Immunother. 2015;11:553–63. https://doi.org/10.1080/21645515.2015.1011562 .
Black S. Safety and effectiveness of MF-59 adjuvanted influenza vaccines in children and adults. Vaccine. 2015;33:B3–5. https://doi.org/10.1016/j.vaccine.2014.11.062 .
Galli G, Medini D, Borgogni E, Zedda L, Bardelli M, Malzone C, et al. Adjuvanted H5N1 vaccine induces early CD4+ T cell response that predicts long-term persistence of protective antibody levels. Proc Natl Acad Sci U S A. 2009;106:3877–82. https://doi.org/10.1073/pnas.0813390106 .
Orsi A, Ansaldi F, De Florentiis D, Ceravolo A, Parodi V, Canepa P, et al. Cross-protection against drifted influenza viruses: Options offered by adjuvanted and intradermal vaccines. Hum Vaccines Immunother. 2013;9:582–90. https://doi.org/10.4161/hv.23239 .
Article CAS Google Scholar
Ansaldi F, Bacilieri S, Durando P, Sticchi L, Valle L, Montomoli E, et al. Cross-protection by MF59 TM -adjuvanted influenza vaccine: Neutralizing and haemagglutination-inhibiting antibody activity against A(H3N2) drifted influenza viruses. Vaccine. 2008;26:1525–9. https://doi.org/10.1016/j.vaccine.2008.01.019 .
Domnich A, Arata L, Amicizia D, Puig-Barberà J, Gasparini R, Panatto D. Effectiveness of MF59-adjuvanted seasonal influenza vaccine in the elderly: A systematic review and meta-analysis. Vaccine. 2017;35:513–20. https://doi.org/10.1016/j.vaccine.2016.12.011 .
van Aalst R, Gravenstein S, Mor V, Mahmud SM, Wilschut J, Postma M, et al. Comparative effectiveness of high dose versus adjuvanted influenza vaccine: A retrospective cohort study. Vaccine. 2020;38:372–9. https://doi.org/10.1016/j.vaccine.2019.09.105 .
Boikos C, Fischer L, O’Brien D, Vasey J, Sylvester GC, Mansi JA. Relative Effectiveness of Adjuvanted Trivalent Inactivated Influenza Vaccine Versus Egg-Derived Quadrivalent Inactivated Influenza Vaccines and High-Dose Trivalent Influenza Vaccine in Preventing Influenza-Related Medical Encounters in US Adults ≥65 Years. Clin Infect Dis. 2021;73:e692–8. https://doi.org/10.1093/cid/ciab152 .
Sanchez L, Matsuoka O, Inoue S, Inoue T, Meng Y, Nakama T, et al. Immunogenicity and safety of high-dose quadrivalent influenza vaccine in Japanese adults ≥65 years of age: a randomized controlled clinical trial. Hum Vaccines Immunother. 2020;16:858–66. https://doi.org/10.1080/21645515.2019.1677437 .
Chang LJ, Meng Y, Janosczyk H, Landolfi V, Talbot HK. Safety and immunogenicity of high-dose quadrivalent influenza vaccine in adults ≥65 years of age: A phase 3 randomized clinical trial. Vaccine. 2019;37:5825–34. https://doi.org/10.1016/j.vaccine.2019.08.016 .
Essink B, Fierro C, Rosen J, Figueroa AL, Zhang B, Verhoeven C, et al. Immunogenicity and safety of MF59-adjuvanted quadrivalent influenza vaccine versus standard and alternate B strain MF59-adjuvanted trivalent influenza vaccines in older adults. Vaccine. 2020;38:242–50. https://doi.org/10.1016/j.vaccine.2019.10.021 .
Michaelis K, Scholz S, Buda S, Garbe E, Harder T, Ledig T, et al. Beschluss und Wissenschaftliche Begründung der Ständigen Impfkommission für die Aktualisierung der Influenza-Impfempfehlung für Personen im Alter von ≥60 Jahren. Epid Bull. 2021;1:3–25.
Joint Committee on Vaccination and Immunisation. Advice on influenza vaccines for 2020/2021. (2019) Available at: https://app.box.com/s/t5ockz9bb6xw6t2mrrzb144njplimfo0/file/529004924372 . Accessed 23 May 2021.
Joint Committee on Vaccination and Immunisation. Advice on influenza vaccines for 2021/2022. (2020)
Grohskopf LA, Alyanak E, Broder KR, Blanton LH, Fry AM, Jernigan DB, et al. Prevention and control of seasonal influenza with vaccines: Recommendations of the advisory committee on immunization practices-United States, 2020-21 influenza season. MMWR Recomm Reports. 2020;69. https://doi.org/10.15585/MMWR.RR6908A1 .
Nguyen-Contant P, Sangster MY, Topham DJ. Squalene-based influenza vaccine adjuvants and their impact on the hemagglutinin-specific b cell response. Pathogens. 2021;10. https://doi.org/10.3390/pathogens10030355 .
Nicholson KG, Abrams KR, Batham S, Clark TW, Hoschler K, Shen Lim W, et al. Immunogenicity and safety of a two-dose schedule of whole-virion and AS03 A-adjuvanted 2009 infl uenza A (H1N1) vaccines: a randomised, multicentre, age-stratifi ed, head-to-head trial. Lancet Infect Dis. 2011;11:91–101. https://doi.org/10.1016/S1473 .
Yang WH, Dionne M, Kyle M, Aggarwal N, Li P, Madariaga M, et al. Long-term immunogenicity of an AS03-adjuvanted influenza A(H1N1)pdm09 vaccine in young and elderly adults: An observer-blind, randomized trial. Vaccine. 2013;31:4389–97. https://doi.org/10.1016/j.vaccine.2013.07.007 .
McElhaney JE, Beran J, Devaster J-M, Esen M, Launay O, Leroux-Roels G, et al. AS03-adjuvanted versus non-adjuvanted inactivated trivalent influenza vaccine against seasonal influenza in elderly people: a phase 3 randomised trial. Lancet Infect Dis. 2013;13:485–96. https://doi.org/10.1016/S1473-3099(13)70046-X .
Tregoning JS, Russell RF, Kinnear E. Adjuvanted influenza vaccines. Hum Vaccines Immunother. 2018;14:550–64. https://doi.org/10.1080/21645515.2017.1415684 .
Petsch B, Schnee M, Vogel AB, Lange E, Hoffmann B, Voss D, et al. Protective efficacy of in vitro synthesized, specific mRNA vaccines against influenza A virus infection. Nat Biotechnol. 2012;30:1210–6. https://doi.org/10.1038/nbt.2436 .
Zimmerman RK, Nowalk MP, Chung J, Jackson ML, Jackson LA, Petrie JG, et al. 2014-2015 Influenza Vaccine Effectiveness in the United States by Vaccine Type. Clin Infect Dis. 2016;63:1564–73. https://doi.org/10.1093/cid/ciw635 .
Xie H, Wan XF, Ye Z, Plant EP, Zhao Y, Xu Y, et al. H3N2 Mismatch of 2014-15 Northern Hemisphere Influenza Vaccines and Head-to-head Comparison between Human and Ferret Antisera derived Antigenic Maps. Sci Rep. 2015;5:1–10. https://doi.org/10.1038/srep15279 .
Belongia EA, Kieke BA, Donahue JG, Greenlee RT, Balish A, Foust A, et al. Effectiveness of inactivated influenza vaccines varied substantially with antigenic match from the 2004-2005 season to the 2006-2007 season. J Infect Dis. 2009;199:159–67. https://doi.org/10.1086/595861 .
Li Z, Zhao Y, Li Y, Chen X. Adjuvantation of influenza vaccines to induce cross-protective immunity. Vaccines. 2021;9:1–22. https://doi.org/10.3390/vaccines9020075 .
Schmidt A, Lapuente D. T cell immunity against influenza: The long way from animal models towards a real-life universal flu vaccine. Viruses. 2021;13. https://doi.org/10.3390/v13020199 .
Estrada LD, Schultz-Cherry S. Development of a universal influenza vaccine. J Immunol. 2019;202:392–8. https://doi.org/10.4049/jimmunol.1801054 .
Nachbagauer R, Palese P. Is a Universal Influenza Virus Vaccine Possible? Annu Rev Med. 2020;71:315–27. https://doi.org/10.1146/annurev-med-120617-041310 .
Wei C-J, Crank MC, Shiver J, Graham BS, Mascola JR, Nabel GJ. Next-generation influenza vaccines: opportunities and challenges. Nat Rev Drug Discov. 2020;19:239–52. https://doi.org/10.1038/s41573-019-0056-x .
Henrichsen J. Six newly recognized types of Streptococcus pneumoniae. J Clin Microbiol. 1995;33:2759–62.
Kim L, McGee L, Tomczyk S, Beall B. Biological and epidemiological features of antibiotic-resistant Streptococcus pneumoniae in pre- and post-conjugate vaccine eras: A United States perspective. Clin Microbiol Rev. 2016;29:525–52. https://doi.org/10.1128/CMR.00058-15 .
Drijkoningen JJC, Rohde GGU. Pneumococcal infection in adults: Burden of disease. Clin Microbiol Infect. 2014;20:45–51. https://doi.org/10.1111/1469-0691.12461 .
Klein EY, Monteforte B, Gupta A, Jiang W, May L, Hsieh YH, et al. The frequency of influenza and bacterial coinfection: a systematic review and meta-analysis. Influenza Other Respi Viruses. 2016;10:394–403. https://doi.org/10.1111/irv.12398 .
Kelly DF, Snape MD, Cutterbuck EA, Green S, Snowden C, Diggle L, et al. CRM197-conjugated serogroup C meningococcal capsular polysaccharide, but not the native polysaccharide, induces persistent antigen-specific memory B cells. Blood. 2006;108:2642–7. https://doi.org/10.1182/blood-2006-01-009282 .
Pollard AJ, Perrett KP, Beverley PC. Maintaining protection agaisnt invasive bacteria with protein-polysaccharide conjugate vaccines. Nat Rev Immunol. 2009;426:422–6 Available at: www.nature.com/reviews/immunol .
Pilishvili T, Lexau C, Farley MM, Hadler J, Harrison LH, Bennett NM, et al. Sustained Reductions in Invasive Pneumococcal Disease in the Era of Conjugate Vaccine. J Infect Dis. 2010;201:32–41. https://doi.org/10.1086/648593 .
Henckaerts I, Goldblatt D, Ashton L, Poolman J. Critical differences between pneumococcal polysaccharide enzyme-linked immunosorbent assays with and without 22F inhibition at low antibody concentrations in pediatric sera. Clin Vaccine Immunol. 2006;13:356–60. https://doi.org/10.1128/CVI.13.3.356-360.2006 .
Song JY, Moseley MA, Burton RL, Nahm MH. Pneumococcal vaccine and opsonic pneumococcal antibody. J Infect Chemother. 2013;19:412–25.
MacIntyre CR, Ridda I, Trent MJ, McIntyre P. Persistence of immunity to conjugate and polysaccharide pneumococcal vaccines in frail, hospitalised older adults in long-term follow up. Vaccine. 2019;37:5016–24. https://doi.org/10.1016/j.vaccine.2019.07.005 .
Park S, Nahm MH. Older adults have a low capacity to opsonize pneumococci due to low igm antibody response to pneumococcal vaccinations. Infect Immun. 2011;79:314–20. https://doi.org/10.1128/IAI.00768-10 .
Adler H, Ferreira DM, Gordon SB, Rylance J. Pneumococcal Capsular Polysaccharide Immunity in the Elderly. Clin Vaccine Immunol. 2017;24:1–18.
Lee H, Nahm MH, Burton R, Kim KH. Immune response in infants to the heptavalent pneumococcal conjugate vaccine against vaccine-related serotypes 6A and 19A. Clin Vaccine Immunol. 2009;16:376–81. https://doi.org/10.1128/CVI.00344-08 .
Oishi T, Ishiwada N, Matsubara K, Nishi J, Chang B, Tamura K, et al. Low opsonic activity to the infecting serotype in pediatric patients with invasive pneumococcal disease. Vaccine. 2013;31:845–9. https://doi.org/10.1016/j.vaccine.2012.11.010 .
Wu YCB, Kipling D, Dunn-Walters DK. Age-related changes in human peripheral blood IGH repertoire following vaccination. Front Immunol. 2012;3:1–12. https://doi.org/10.3389/fimmu.2012.00193 .
Kolibab K, Smithson SL, Shriner AK, Khuder S, Romero-Steiner S, Carlone GM, et al. Immune response to pneumococcal polysaccharides 4 and 14 in elderly and young adults. I Antibody concentrations, avidity and functional activity. Immun Ageing. 2005;2:1–9. https://doi.org/10.1186/1742-4933-2-10 .
Romero-Steiner S, Musher DM, Cetron MS, Pais LB, Groover JE, Carlone GM. Reduction in Functional Antibody Activity Against Streptococcus pneumoniae in Vaccinated Elderly Individuals Highly Correlates with Decreased IgG Antibody Avidity. Clin Infect Dis. 1999;29:281–8. https://doi.org/10.1086/520200 .
de Roux A, Schmöele-Thoma B, Siber GR, Hackell JG, Kuhnke A, Ahlers N, et al. Comparison of Pneumococcal Conjugate Polysaccharide and Free Polysaccharide Vaccines in Elderly Adults: Conjugate Vaccine Elicits Improved Antibacterial Immune Responses and Immunological Memory. Clin Infect Dis. 2008;46:1015–23. https://doi.org/10.1086/529142 .
Ridda I, MacIntyre CR, Lindley R, Gao Z, Sullivan JS, Yuan FF, et al. Immunological responses to pneumococcal vaccine in frail older people. Vaccine. 2009;27:1628–36. https://doi.org/10.1016/j.vaccine.2008.11.098 .
MacIntyre CR, Ridda I, Gao Z, Moa AM, McIntyre PB, Sullivan JS, et al. A randomized clinical trial of the immunogenicity of 7-valent pneumococcal conjugate vaccine compared to 23-valent polysaccharide vaccine in frail, hospitalized elderly. PLoS One. 2014;9:e94578. https://doi.org/10.1371/journal.pone.0094578 .
Miernyk KM, Butler JC, Bulkow LR, Singleton RJ, Hennessy TW, Dentinger CM, et al. Immunogenicity and Reactogenicity of Pneumococcal Polysaccharide and Conjugate Vaccines in Alaska Native Adults 55–70 Years of Age. Clin Infect Dis. 2009;49:241–8. https://doi.org/10.1086/599824 .
Vadlamudi NK, Parhar K, Altre Malana KL, Kang A, Marra F. Immunogenicity and safety of the 13-valent pneumococcal conjugate vaccine compared to 23-valent pneumococcal polysaccharide in immunocompetent adults: A systematic review and meta-analysis. Vaccine. 2019;37:1021–9. https://doi.org/10.1016/j.vaccine.2019.01.014 .
Marra F, Vadlamudi NK. Efficacy and safety of the pneumococcal conjugate-13 valent vaccine in adults. Aging Dis. 2019;10:404–18. https://doi.org/10.14336/AD.2018.0512 .
Falkenhorst G, Remschmidt C, Harder T, Hummers-Pradier E, Wichmann O, Bogdan C. Effectiveness of the 23-valent pneumococcal polysaccharide vaccine (ppv23) against pneumococcal disease in the elderly: Systematic review and meta-analysis. PLoS One. 2017;12:1–18. https://doi.org/10.1371/journal.pone.0169368 .
Diao WQ, Shen N, Yu PX, Liu BB, He B. Efficacy of 23-valent pneumococcal polysaccharide vaccine in preventing community-acquired pneumonia among immunocompetent adults: A systematic review and meta-analysis of randomized trials. Vaccine. 2016;34:1496–503. https://doi.org/10.1016/j.vaccine.2016.02.023 .
Kraicer-Melamed H, O’Donnell S, Quach C. The effectiveness of pneumococcal polysaccharide vaccine 23 (PPV23) in the general population of 50 years of age and older: A systematic review and meta-analysis. Vaccine. 2016;34:1540–50. https://doi.org/10.1016/j.vaccine.2016.06.045 .
Schiffner-Rohe J, Witt A, Hemmerling J, Von Eiff C, Leverkus FW. Efficacy of PPV23 in preventing pneumococcal pneumonia in adults at increased risk - A systematic review and meta-analysis. PLoS One. 2016;11:1–21. https://doi.org/10.1371/journal.pone.0146338 .
Moberley S, Holden J, Tatham T, Andrews R. Vaccines for preventing pneumococcal infection in adults. Cochrane Database Syst Rev. 2013;1:CD000422.
Bonten MJM, Huijts SM, Bolkenbaas M, Webber C, Patterson S, Gault S, et al. Polysaccharide conjugate vaccine against pneumococcal pneumonia in adults. N Engl J Med. 2015;372:1114–25. https://doi.org/10.1056/NEJMoa1408544 .
Richmond P, Kaczmarski E, Borrow R, Findlow J, Clark S, McCann R, et al. Meningococcal C Polysaccharide Vaccine Induces Immunologic Hyporesponsiveness in Adults That Is Overcome by Meningococcal C Conjugate Vaccine. J Infect Dis. 2000;181:761–4. https://doi.org/10.1086/315284 .
Matanock A, Lee G, Gierke R, Kobayashi M, Leidner A, Pilishvili T. Use of 13-Valent Pneumococcal Conjugate Vaccine and 23-Valent Pneumococcal Polysaccharide Vaccine Among Adults Aged ≥65 Years: Updated Recommendations of the Advisory Committee on Immunization Practices. MMWR Morb Mortal Wkly Rep. 2019;68:1069–75. https://doi.org/10.15585/mmwr.mm6846a5 .
Kaplan A, Aresnault P, Aw B, Brown V, Fox G, Grossman R, et al. Vaccine strategies for prevention of community-acquired pneumonia in Canada. Candadian Fam Physician. 2019;65:625–33 Available at: https://pubmed.ncbi.nlm.nih.gov/31515311/ .
Vila-Córcoles A, Ochoa-Gondar O, de Diego C, Satué E, Vila-Rovira A, Aragón M. Pneumococcal vaccination coverages by age, sex and specific underlying risk conditions among middle-aged and older adults in Catalonia, Spain, 2017. Eurosurveillance. 2019;24:1–9. https://doi.org/10.2807/1560-7917.ES.2019.24.29.1800446 .
Norris T, Vahratian A, Cohen RA. Vaccination Coverage Among Adults Aged 65 and Over: United States, 2015. NCHS Data Brief (2017)1–8.
Black CL, Williams WW, Warnock R, Pilishvili T, Kim D, Kelman JA. Pneumococcal vaccination among medicare beneficiaries occurring after the advisory committee on immunization practices recommendation for routine use of 13-valent pneumococcal conjugate vaccine and 23-valent pneumococcal polysaccharide vaccine for adults. Morb Mortal Wkly Rep. 2017;66:728–33. https://doi.org/10.15585/mmwr.mm6627a4 .
Stacey HL, Rosen J, Peterson JT, Williams-Diaz A, Gakhar V, Sterling TM, et al. Safety and immunogenicity of 15-valent pneumococcal conjugate vaccine (PCV-15) compared to PCV-13 in healthy older adults. Hum Vaccines Immunother. 2019;15:530–9. https://doi.org/10.1080/21645515.2018.1532249 .
Hurley D, Griffin C, Young M, Scott DA, Pride MW, Scully IL, et al. Safety, Tolerability, and Immunogenicity of a 20-Valent Pneumococcal Conjugate Vaccine (PCV20) in Adults 60 to 64 Years of Age. Clin Infect Dis. 2020:1–9. https://doi.org/10.1093/cid/ciaa1045 .
Merck. Merck announces US FDA approval of Vaxneuvance TM (pneumococcal 15-valent conjugate vaccine) for the prevention of invasive pneumococcal disease in adults 18 years and older caused by 15 serotypes. (2021) Available at: https://www.businesswire.com/news/home/20210716005480/en/Merck-Announces-U.S.-FDA-Approval-of-VAXNEUVANCE TM -Pneumococcal-15-valent-Conjugate-Vaccine-for-the-Prevention-of-Invasive-Pneumococcal-Disease-in-Adults-18-Years-and-Older-Caused-by-15-Serotypes . Accessed 29 July 2021.
Flebbe LM, Braley-Mullen H. Immunopotentiating effects of the adjuvants SGP and Quil A. Cell Immunol. 1986;99:119–27. https://doi.org/10.1016/0008-8749(86)90221-2 .
Wuorimaa T, Dagan R, Eskola J, Janco J, Åhman H, Leroy O, et al. Tolerability and immunogenicity of an eleven-valent pneumococcal conjugate vaccine in healthy toddlers. Pediatr Infect Dis J. 2001;20. https://doi.org/10.1097/00006454-200103000-00011 .
Taillardet M, Haffar G, Mondière P, Asensio MJ, Pléau-Pison T, Burdin N, et al. Toll-like receptor agonists allow generation of long-lasting antipneumococcal humoral immunity in response to a plain polysaccharidic vaccine. J Infect Dis. 2010;202:470–9. https://doi.org/10.1086/653739 .
Olafsdottir TA, Lingnau K, Nagy E, Jonsdottir I. IC31®, a two-component novel adjuvant mixed with a conjugate vaccine enhances protective immunity against pneumococcal disease in neonatal mice. Scand J Immunol. 2009;69:194–202. https://doi.org/10.1111/j.1365-3083.2008.02225.x .
Phipps JP, Haas KM. An Adjuvant That Increases Protective Antibody Responses to Polysaccharide Antigens and Enables Recall Responses. J Infect Dis. 2019;219:323–34. https://doi.org/10.1093/infdis/jiy506 .
Campos IB, Herd M, Moffitt KL, Lu YJ, Darrieux M, Malley R, et al. IL-17A and complement contribute to killing of pneumococci following immunization with a pneumococcal whole cell vaccine. Vaccine. 2017;35:1306–15. https://doi.org/10.1016/j.vaccine.2017.01.030 .
Lu YJ, Yadav P, Clements JD, Forte S, Srivastava A, Thompson CM, et al. Options for inactivation, adjuvant, and route of topical administration of a killed, unencapsulated pneumococcal whole-cell vaccine. Clin Vaccine Immunol. 2010;17:1005–12. https://doi.org/10.1128/CVI.00036-10 .
Lu YJ, Leite L, Gonçalves VM, de Oliveira Dias W, Liberman C, Fratelli F, et al. GMP-grade pneumococcal whole-cell vaccine injected subcutaneously protects mice from nasopharyngeal colonization and fatal aspiration-sepsis. Vaccine. 2010;28:7468–75. https://doi.org/10.1016/j.vaccine.2010.09.031 .
Campo JJ, Le TQ, Pablo JV, Hung C, Teng AA, Tettelin H, et al. Panproteome-wide analysis of antibody responses to whole cell pneumococcal vaccination. Elife. 2018;7:e37015. https://doi.org/10.7554/eLife.37015 .
Keech CA, Morrison R, Anderson P, Tate A, Flores J, Goldblatt D, et al. Trial to Evaluate the Safety and Immunogenicity of Inactivated Streptococcus pneumoniae Whole-cell Vaccine in Adults. Pediatr Infect Dis J. 2019) Epub ahead of print. https://doi.org/10.1097/INF.0000000000002567 .
Pichichero ME, Khan MN, Xu Q. Next generation protein based Streptococcus pneumoniae vaccines. Hum Vaccines Immunother. 2016;12:194–205. https://doi.org/10.1080/21645515.2015.1052198 .
Pichichero ME. Pneumococcal whole-cell and protein-based vaccines: changing the paradigm. Expert Rev Vaccines. 2017;16:1181–90. https://doi.org/10.1080/14760584.2017.1393335 .
Lagousi T, Basdeki P, Routsias J, Spoulou V. Novel protein-based pneumococcal vaccines: Assessing the use of distinct protein fragments instead of full-length proteins as vaccine antigens. Vaccines. 2019;7:1–18. https://doi.org/10.3390/vaccines7010009 .
Masomian M, Ahmad Z, Gew LT, Poh CL. Development of next generation streptococcus pneumoniae vaccines conferring broad protection. Vaccines. 2020;8. https://doi.org/10.3390/vaccines8010132 .
Kawai K, Gebremeskel BG, Acosta CJ. Systematic review of incidence and complications of herpes zoster: Towards a global perspective. BMJ Open. 2014;4:e004833. https://doi.org/10.1136/bmjopen-2014-004833 .
Johnson RW, Rice ASC. Postherpetic neuralgia. N Engl J Med. 2014;371:1526–33. https://doi.org/10.1056/NEJMcp1403062 .
Opstelten W, McElhaney J, Weinberger B, Oaklander AL, Johnson RW. The impact of varicella zoster virus: Chronic pain. J Clin Virol. 2010;48:S8–S13. https://doi.org/10.1016/S1386-6532(10)70003-2 .
Yawn BP, Gilden D. The global epidemiology of herpes zoster. Neurology. 2013;81:928–30. https://doi.org/10.1212/wnl.0b013e3182a3516e .
Garçon N, Van Mechelen M. Recent clinical experience with vaccines using MPL- and QS-21-containing Adjuvant Systems. Expert Rev Vaccines. 2011. https://doi.org/10.1586/erv.11.29 .
Del Giudice G, Rappuoli R, Didierlaurent AM. Correlates of adjuvanticity: A review on adjuvants in licensed vaccines. Semin Immunol. 2018;39:14–21. https://doi.org/10.1016/j.smim.2018.05.001 .
Detienne S, Welsby I, Collignon C, Wouters S, Coccia M, Delhaye S, et al. Central role of CD169+ lymph node resident macrophages in the adjuvanticity of the QS-21 component of AS01. Sci Rep. 2016;6:1–14. https://doi.org/10.1038/srep39475 .
Welsby I, Detienne S, N’Kuli F, Thomas S, Wouters S, Bechtold V, et al. Lysosome-dependent activation of human dendritic cells by the vaccine adjuvant QS-21. Front Immunol. 2017;7:663. https://doi.org/10.3389/fimmu.2016.00663 .
Lacaille-Dubois MA. Updated insights into the mechanism of action and clinical profile of the immunoadjuvant QS-21: A review. Phytomedicine. 2019;60. https://doi.org/10.1016/j.phymed.2019.152905 .
Didierlaurent AM, Laupèze B, Di Pasquale A, Hergli N, Collignon C, Garçon N. Adjuvant system AS01: helping to overcome the challenges of modern vaccines. Expert Rev Vaccines. 2017;16:55–63. https://doi.org/10.1080/14760584.2016.1213632 .
Levin MJ, Oxman MN, Zhang JH, Johnson GR, Stanley H, Hayward AR, et al. Varicella-Zoster Virus–Specific Immune Responses in Elderly Recipients of a Herpes Zoster Vaccine. J Infect Dis. 2008;197:825–35. https://doi.org/10.1086/528696 .
Schwarz TF, Volpe S, Catteau G, Chlibek R, David MP, Richardus JH, et al. Persistence of immune response to an adjuvanted varicella-zoster virus subunit vaccine for up to year nine in older adults. Hum Vaccines Immunother. 2018;14:1370–7. https://doi.org/10.1080/21645515.2018.1442162 .
Chlibek R, Bayas JM, Collins H, De La Pinta MLR, Ledent E, Mols JF, et al. Safety and immunogenicity of an ASO1 -adjuvanted varicella-zoster virus subunit candidate vaccine against herpes zoster in adults ≥50 years of age. J Infect Dis. 2013;208:1953–61. https://doi.org/10.1093/infdis/jit365 .
Chlibek R, Smetana J, Pauksens K, Rombo L, Van den Hoek JAR, Richardus JH, et al. Safety and immunogenicity of three different formulations of an adjuvanted varicella-zoster virus subunit candidate vaccine in older adults: A phase II, randomized, controlled study. Vaccine. 2014;32:1745–53. https://doi.org/10.1016/j.vaccine.2014.01.019 .
Leroux-Roels I, Leroux-Roels G, Clement F, Vandepapelière P, Vassilev V, Ledent E, et al. A phase 1/2 clinical trial evaluating safety and immunogenicity of a varicella zoster glycoprotein e subunit vaccine candidate in young and older adults. J Infect Dis. 2012;206:1280–90. https://doi.org/10.1093/infdis/jis497 .
Cunningham AL, Heineman TC, Lal H, Godeaux O, Chlibek R, Hwang SJ, et al. Immune responses to a recombinant glycoprotein e herpes zoster vaccine in adults aged 50 years or older. J Infect Dis. 2018;217:1750–60. https://doi.org/10.1093/infdis/jiy095 .
Levin MJ, Kroehl ME, Johnson MJ, Hammes A, Reinhold D, Lang N, et al. Th1 memory differentiates recombinant from live herpes zoster vaccines. J Clin Invest. 2018;128:4429–40. https://doi.org/10.1172/JCI121484 .
Schmid DS, Miao C, Leung J, Johnson M, Weinberg A, Levin MJ. Comparative Antibody Responses to the Live-Attenuated and Recombinant Herpes Zoster Vaccines. J Virol. 2021;95. https://doi.org/10.1128/jvi.00240-21 .
Oxman MN, Levin MJ, Johnson GR, Schmader KE, Straus SE, Gelb LD, et al. A Vaccine to Prevent Herpse Zoster and Postherpetic Neuralgia in Older Adults. N Engl J Med. 2005;352:2271–84.
Schmader KE, Levin MJ, Gnann JW, McNeil SA, Vesikari T, Betts RF, et al. Efficacy, safety, and tolerability of herpes zoster vaccine in persons aged 50-59 years. Clin Infect Dis. 2012;54:922–8. https://doi.org/10.1093/cid/cir970 .
Morrison VA, Johnson GR, Schmader KE, Levin MJ, Zhang JH, Looney DJ, et al. Long-term persistence of zoster vaccine efficacy. Clin Infect Dis. 2015;60:900–9. https://doi.org/10.1093/cid/ciu918 .
Weinberg A, Popmihajlov Z, Schmader KE, Johnson MJ, Caldas Y, Salazar AT, et al. Persistence of Varicella-Zoster Virus Cell-Mediated Immunity after the Administration of a Second Dose of Live Herpes Zoster Vaccine. J Infect Dis. 2019;219:335–8. https://doi.org/10.1093/infdis/jiy514 .
Cunningham AL, Lal H, Kovac M, Chlibek R, Hwang SJ, Díez-Domingo J, et al. Efficacy of the herpes zoster subunit vaccine in adults 70 years of age or older. N Engl J Med. 2016;375:1019–32. https://doi.org/10.1056/NEJMoa1603800 .
Lal H, Cunningham AL, Godeaux O, Chlibek R, Diez-Domingo J, Hwang SJ, et al. Efficacy of an adjuvanted herpes zoster subunit vaccine in older adults. N Engl J Med. 2015;372:2087–96. https://doi.org/10.1056/NEJMoa1501184 .
Boutry C, Hastie A, Diez-Domingo J, Tinoco JC, Yu C-J, Andrews C, et al. The Adjuvanted Recombinant Zoster Vaccine Confers Long-term Protection Against Herpes Zoster: Interim Results of an Extension Study of the Pivotal Phase III Clinical Trials (ZOE-50 and ZOE-70). Clin Infect Dis. 2021. https://doi.org/10.1093/cid/ciab629 .
Curran D, Kim JH, Matthews S, Dessart C, Levin MJ, Oostvogels L, et al. Recombinant Zoster Vaccine Is Efficacious and Safe in Frail Individuals. J Am Geriatr Soc. 2021;69:744–52. https://doi.org/10.1111/jgs.16917 .
Izurieta HS, Wu X, Forshee R, Lu Y, Sung H-M, Ehrlich Agger P, et al. Recombinant Zoster Vaccine (Shingrix) real-world effectiveness in the first two years post- licensure. Clin Infect Dis. 2021:ciab125. https://doi.org/10.1093/cid/ciab125 .
Vink P, Ramon Torrell JM, Sanchez Fructuoso A, Kim S-J, Kim S, Zaltzman J, et al. Immunogenicity and Safety of the Adjuvanted Recombinant Zoster Vaccine in Chronically Immunosuppressed Adults Following Renal Transplant: a Phase III, Randomized Clinical Trial. Clin Infect Dis. 2019;70:181–90. https://doi.org/10.1093/cid/ciz177 .
Article CAS PubMed Central Google Scholar
Berkowitz EM, Moyle G, Stellbrink HJ, Schürmann D, Kegg S, Stoll M, et al. Safety and immunogenicity of an adjuvanted herpes zoster subunit candidate vaccine in HIV-infected adults: A phase 1/2a randomized, placebo-controlled study. J Infect Dis. 2015;211:1279–87. https://doi.org/10.1093/infdis/jiu606 .
Dagnew AF, Ilhan O, Lee WS, Woszczyk D, Kwak JY, Bowcock S, et al. Immunogenicity and safety of the adjuvanted recombinant zoster vaccine in adults with haematological malignancies: a phase 3, randomised, clinical trial and post-hoc efficacy analysis. Lancet Infect Dis. 2019;19:988–1000. https://doi.org/10.1016/S1473-3099(19)30163-X .
Winston DJ, Mullane KM, Cornely OA, Boeckh MJ, Brown JW, Pergam SA, et al. Inactivated varicella zoster vaccine in autologous haemopoietic stem-cell transplant recipients: an international, multicentre, randomised, double-blind, placebo-controlled trial. Lancet. 2018;391:2116–27. https://doi.org/10.1016/S0140-6736(18)30631-7 .
Booth A, Reed AB, Ponzo S, Yassaee A, Aral M, Plans D, et al. Population risk factors for severe disease and mortality in COVID-19: A global systematic review and meta-analysis. PLoS One. 2021;16:e0247461. https://doi.org/10.1371/journal.pone.0247461 .
Pijls BG, Jolani S, Atherley A, Derckx RT, Dijkstra JIR, Franssen GHL, et al. Demographic risk factors for COVID-19 infection, severity, ICU admission and death: A meta-analysis of 59 studies. BMJ Open. 2021;11:e044640. https://doi.org/10.1136/bmjopen-2020-044640 .
Flook M, Jackson C, Vasileiou E, Simpson CR, Muckian MD, Agrawal U, et al. Informing the public health response to COVID-19: a systematic review of risk factors for disease, severity, and mortality. BMC Infect Dis. 2021;21:342. https://doi.org/10.1186/s12879-021-05992-1 .
Korompoki E, Gavriatopoulou M, Hicklen RS, Ntanasis-Stathopoulos I, Kastritis E, Fotiou D, et al. Epidemiology and organ specific sequelae of post-acute COVID19: A narrative review. J Infect. 2021. https://doi.org/10.1016/j.jinf.2021.05.004 .
Nalbandian A, Sehgal K, Gupta A, Madhavan MV, McGroder C, Stevens JS, et al. Post-acute COVID-19 syndrome. Nat Med. 2021;27:601–15. https://doi.org/10.1038/s41591-021-01283-z .
World Health Organisation. COVID-19 vaccine tracker and landscape. (2021) Available at: https://www.who.int/publications/m/item/draft-landscape-of-covid-19-candidate-vaccines
McDonald I, Murray SM, Reynolds CJ, Altmann DM, Boyton RJ. Comparative systematic review and meta-analysis of reactogenicity, immunogenicity and efficacy of vaccines against SARS-CoV-2. npj Vaccines. 2021:6, 1–14. https://doi.org/10.1038/s41541-021-00336-1 .
Anderson EJ, Rouphael NG, Widge AT, Jackson LA, Roberts PC, Makhene M, et al. Safety and Immunogenicity of SARS-CoV-2 mRNA-1273 Vaccine in Older Adults. N Engl J Med. 2020;383:2427–38. https://doi.org/10.1056/nejmoa2028436 .
Walsh EE, Frenck RW, Falsey AR, Kitchin N, Absalon J, Gurtman A, et al. Safety and Immunogenicity of Two RNA-Based Covid-19 Vaccine Candidates. N Engl J Med. 2020;383:2439–50. https://doi.org/10.1056/nejmoa2027906 .
Sadoff J, Gray G, Vandebosch A, Cárdenas V, Shukarev G, Grinsztejn B, et al. Safety and Efficacy of Single-Dose Ad26.COV2.S Vaccine against Covid-19. N Engl J Med. 2021:1–15. https://doi.org/10.1056/nejmoa2101544 .
Ramasamy MN, Aley PK, Angus B, Babbage G, Belij-Rammerstorfer S, Berry L, et al. Safety and immunogenicity of ChAdOx1 nCoV-19 vaccine administered in a prime-boost regimen in young and old adults ( COV002 ): a single-blind , randomised , controlled , phase 2 / 3 trial. Lancet. 2021;396:1979–93.
Collier DA, Ferreira IATM, Kotagiri P, Datir RP, Lim EY, Touizer E, et al. Age-related immune response heterogeneity to SARS-CoV-2 vaccine BNT162b2. Nature. 2021;596:417–22. https://doi.org/10.1038/s41586-021-03739-1 .
Baden LR, El Sahly HM, Essink B, Kotloff K, Frey S, Novak R, et al. Efficacy and Safety of the mRNA-1273 SARS-CoV-2 Vaccine. N Engl J Med. 2021;384:403–16. https://doi.org/10.1056/nejmoa2035389 .
Polack FP, Thomas SJ, Kitchin N, Absalon J, Gurtman A, Lockhart S, et al. Safety and Efficacy of the BNT162b2 mRNA Covid-19 Vaccine. N Engl J Med. 2020;383:2603–15. https://doi.org/10.1056/nejmoa2034577 .
European Medicines Agency. Assessment report: COVID-19 Vaccine AstraZeneca. (2021) Available at: https://www.ema.europa.eu/en/documents/assessment-report/vaxzevria-previously-covid-19-vaccine-astrazeneca-epar-public-assessment-report_en.pdf
Shimabukuro TT, Cole M, Su JR. Reports of Anaphylaxis after Receipt of mRNA COVID-19 Vaccines in the US-December 14, 2020-January 18, 2021. JAMA - J Am Med Assoc. 2021;325:1101–2. https://doi.org/10.1001/jama.2021.1967 .
Banerji A, Wickner PG, Saff R, Stone CA, Robinson LB, Long AA, et al. mRNA Vaccines to Prevent COVID-19 Disease and Reported Allergic Reactions: Current Evidence and Suggested Approach. J Allergy Clin Immunol Pract. 2021;9:1423–37. https://doi.org/10.1016/j.jaip.2020.12.047 .
Cines DB, Bussel JB. SARS-CoV-2 Vaccine–Induced Immune Thrombotic Thrombocytopenia. N Engl J Med. 2021. https://doi.org/10.1056/nejme2106315 .
Montgomery J, Ryan M, Engler R, Hoffman D, McClenathan B, Collins L, et al. Myocarditis Following Immunization With mRNA COVID-19 Vaccines in Members of the US Military. JAMA Cardiol. 2021;92134:6–10. https://doi.org/10.1001/jamacardio.2021.2833 .
European Medicines Agency. Assessment report: COVID-19 Vaccine Moderna. (2021) Available at: https://www.ema.europa.eu/en/documents/assessment-report/spikevax-previously-covid-19-vaccine-moderna-epar-public-assessment-report_en.pdf . Accessed 2 Aug 2021.
Voysey M, Ann S, Clemens C, Madhi SA, Weckx LY, Folegatti PM, et al. Safety and efficacy of the ChAdOx1 nCoV-19 vaccine ( AZD1222 ) against SARS-CoV-2 : an interim analysis of four randomised controlled trials in Brazil , South Africa , and the UK. Lancet. 2020:1–13.
News Releases. Investigational AstraZeneca vaccine prevents COVID-19. (2021) Available at: https://www.nih.gov/news-events/news-releases/investigational-astrazeneca-vaccine-prevents-covid-19 . Accessed 4 April 2021.
Hall VJ, Foulkes S, Saei A, Andrews N, Oguti B, Charlett A, et al. Effectiveness of BNT162b2 mRNA Vaccine Against Infection and COVID-19 Vaccine Coverage in Healthcare Workers in England, Multicentre Prospective Cohort Study (the SIREN Study). SSRN Electron J. 2021. https://doi.org/10.2139/ssrn.3790399 .
Thompson MG, Burgess JL, Naleway AL, Tyner HL, Yoon SK, Meece J, et al. Interim Estimates of Vaccine Effectiveness of BNT162b2 and mRNA-1273 COVID-19 Vaccines in Preventing SARS-CoV-2 Infection Among Health Care Personnel, First Responders, and Other Essential and Frontline Workers — Eight U.S. Locations, December 2020–March. MMWR Morb Mortal Wkly Rep. 2021;70:495–500. https://doi.org/10.15585/mmwr.mm7013e3 .
Haas EJ, Angulo FJ, McLaughlin JM, Anis E, Singer SR, Khan F, et al. Impact and effectiveness of mRNA BNT162b2 vaccine against SARS-CoV-2 infections and COVID-19 cases, hospitalisations, and deaths following a nationwide vaccination campaign in Israel: an observational study using national surveillance data. Lancet. 2021;397:1819–29. https://doi.org/10.1016/S0140-6736(21)00947-8 .
Moutsen-Helms IR, Emborg H-D, Nielsen J, Finderup Nielsen K, Grove Krause T, Mølbak K, et al. Vaccine effectiveness after 1st and 2nd dose of the BNT162b2 mRNA Covid-19 Vaccine in long-term care facility residents and healthcare workers – a Danish cohort study. medRxiv. 2021. https://doi.org/10.1101/2021.03.08.21252200 .
Mazagatos C, Monge S, Olmedo C, Vega L, Gallego P, Martin-Merino E, et al. Effectiveness of mRNA COVID-19 vaccines in preventing SARS-CoV-2 infections and COVID-19 hospitalisations and deaths in elderly long-term care facility residents, Spain, weeks 53 2020 to 13 2021. Eurosurveillance. 2021;26:1–6. https://doi.org/10.2807/1560-7917.ES.2021.26.24.2100452 .
Harder T, Koch J, Vygen-Bonnet S, Külper-Schiek W, Pilic A, Reda S, et al. Efficacy and effectiveness of COVID-19 vaccines against SARS-CoV-2 infection: interim results of a living systematic review, 1 January to 14 May 2021. Euro Surveill. 2021;26:1–9. https://doi.org/10.2807/1560-7917.ES.2021.26.28.2100563 .
Voysey M, Costa Clemens SA, Madhi SA, Weckx LY, Folegatti PM, Aley PK, et al. Single-dose administration and the influence of the timing of the booster dose on immunogenicity and efficacy of ChAdOx1 nCoV-19 (AZD1222) vaccine: a pooled analysis of four randomised trials. Lancet. 2021;19:1–11. https://doi.org/10.1016/S0140-6736(21)00432-3 .
Parry H, Bruton R, Stephens C, Brown K, Amirthalingam G, Hallis B, et al. Extended interval BNT162b2 vaccination enhances peak antibody generation in older people. medRxiv. 2021. https://doi.org/10.1101/2021.05.15.21257017 .
Lewis D. The case is growing for mix-and-match COVID vaccines. Nature. 2021;595:344–5.
Doria-Rose NA, Suthar MS. Antibody Persistence through 6 Months after the Second Dose of mRNA-1273 Vaccine for Covid-19. N Engl J Med. 2021;384.
Mateus J, Dan JM, Zhang Z, Moderbacher CR, Lammers M, Goodwin B, Sette A, Crotty S, Weiskopf D. Low dose mRNA-1273 COVID-19 vaccine generates durable T cell memory and antibodies enhanced by pre-existing crossreactive T cell memory. medRxiv (2021) 2021.06.30.21259787. Available at: https://www.medrxiv.org/content/10.1101/2021.06.30.21259787v1 , https://www.medrxiv.org/content/10.1101/2021.06.30.21259787v1.abstract
Ministry of Health. Decline in Vaccine Effectiveness Against Infection and Symptomatic Illness. (2021) Available at: https://www.gov.il/en/departments/news/05072021-03 . Accessed 26 July 2021.
Lopez Bernal J, Andrews N, Gower C, Gallagher E, Simmons R, Thelwall S, et al. Effectiveness of Covid-19 Vaccines against the B.1.617.2 (Delta) Variant. N Engl J Med. 2021;385:585–94. https://doi.org/10.1056/nejmoa2108891 .
Massare MJ, Patel N, Zou B, Maciejewski S, Flores R, Guebre-Xabier M, et al. Combination Respiratory Vaccine Containing Recombinant SARS-CoV-2 Spike and Quadrivalent Seasonal Influenza Hemagglutinin Nanoparticles with Matrix-M Adjuvan. bioRxiv. 2021. https://doi.org/10.1101/2021.05.05.442782 .
Kaml M, Weiskirchner I, Keller M, Luft T, Hoster E, Hasford J, et al. Booster vaccination in the elderly: their success depends on the vaccine type applied earlier in life as well as on pre-vaccination antibody titers. Vaccine. 2006;24:6808–11.
Weinberger B, Schirmer M, Matteucci GR, Siebert U, Fuchs D, Grubeck-Loebenstein B. Recall responses to tetanus and diphtheria vaccination are frequently insufficient in elderly persons. PLoSOne. 2013;8:e82967.
Bayas JM, Vilella A, Bertran MJ, Vidal J, Batalla J, Asenjo MA, et al. Immunogenicity and reactogenicity of the adult tetanus-diphtheria vaccine: How many doses are necessary? Epidemiol Infect. 2001;127:451–60. https://doi.org/10.1017/S095026880100629X .
Van Damme P, Burgess M. Immunogenicity of a combined diphtheria-tetanus-acellular pertussis vaccine in adults. Vaccine. 2004;22:305–8. https://doi.org/10.1016/j.vaccine.2003.08.012 .
Launay O, Toneatti C, Bernède C, Njamkepo E, Petitprez K, Leblond A, et al. Antibodies to tetanus, diphtheria and pertussis among healthy adults vaccinated according to the French vaccination recommendations. Hum Vaccin. 2009;5:341–6. https://doi.org/10.4161/hv.5.5.7575 .
Grasse M, Meryk A, Schirmer M, Grubeck-Loebenstein B, Weinberger B. Booster vaccination against tetanus and diphtheria: insufficient protection against diphtheria in young and elderly adults. Immun Ageing. 2016;13:26. https://doi.org/10.1186/s12979-016-0081-0 .
Weinberger B, Keller M, Putzer C, Breitenberger D, Koller B, Fiegl S, et al. Protection against Tetanus and Diphtheria in Europe: The impact of age, gender and country of origin based on data from the MARK-AGE Study. Exp Gerontol. 2018;105:109–12. https://doi.org/10.1016/j.exger.2017.08.037 .
Weinberger B. Adult vaccination against tetanus and diphtheria: the European perspective. Clin Exp Immunol. 2017;187:93–9. https://doi.org/10.1111/cei.12822 .
Weinberger B, Keller M, Fischer KH, Stiasny K, Neuner C, Heinz FX, et al. Decreased antibody titers and booster responses in tick-borne encephalitis vaccinees aged 50-90 years. Vaccine. 2010. https://doi.org/10.1016/j.vaccine.2010.03.024 .
Stiasny K, Aberle JH, Keller M, Grubeck-Loebenstein B, Heinz FX. Age affects quantity but not quality of antibody responses after vaccination with an inactivated flavivirus vaccine against tick-borne encephalitis. PLoS One. 2012. https://doi.org/10.1371/journal.pone.0034145 .
Ridda I, Yin JK, King C, Raina MacIntyre C, McIntyre P. The importance of pertussis in older adults: a growing case for reviewing vaccination strategy in the elderly. Vaccine. 2012;30:6745–52. https://doi.org/10.1016/j.vaccine.2012.08.079 .
Gil A, Oyagüez I, Carrasco P, González A. Hospital admissions for pertussis in Spain, 1995-1998. Vaccine. 2001;19:4791–4. https://doi.org/10.1016/s0264-410x(01)00213-4 .
Rendi-Wagner P, Tobias J, Moerman L, Goren S, Bassal R, Green M, et al. The seroepidemiology of Bordetella pertussis in Israel--Estimate of incidence of infection. Vaccine. 2010;28:3285–90. https://doi.org/10.1016/j.vaccine.2010.02.104 .
Halperin SA, Scheifele D, De Serres G, Noya F, Meekison W, Zickler P, et al. Immune responses in adults to revaccination with a tetanus toxoid, reduced diphtheria toxoid, and acellular pertussis vaccine 10 years after a previous dose. Vaccine. 2012;30:974–82. https://doi.org/10.1016/j.vaccine.2011.11.035 .
Taylor DN, Pollard RA, Blake PA. Typhoid in the United States and the risk to the international traveler. J Infect Dis. 1983;148:599–602. https://doi.org/10.1093/infdis/148.3.599 .
Hennessy S, Liu Z, Tsai TF, Strom BL, Wan CM, Liu HL, et al. Effectiveness of live-attenuated Japanese encephalitis vaccine (SA14-14-2): a case-control study. Lancet (London, England). 1996;347:1583–6. https://doi.org/10.1016/s0140-6736(96)91075-2 .
Jilg W. “Vaccines for Older Travelers.,” in Interdisciplinary topics in gerontology and geriatrics (Interdiscip Top Gerontol Geriatr). 2020;43:158–181.
Ecarnot F, Maggi S, Michel J-P, Veronese N, Rossanese A. Vaccines and Senior Travellers. Front Aging. 2021;2:1–17. https://doi.org/10.3389/fragi.2021.677907 .
Haynes L, Eaton SM, Burns EM, Randall TD, Swain SL. CD4 T cell memory derived from young naive cells functions well into old age, but memory generated from aged naive cells functions poorly. Proc Natl Acad Sci U S A. 2003;100:15053–8. https://doi.org/10.1073/pnas.2433717100 .
Haynes L. The effect of aging on cognate function and development of immune memory. Curr Opin Immunol. 2005;17:476–9. https://doi.org/10.1016/j.coi.2005.07.003 .
Wolters B, Junge U, Dziuba S, Roggendorf M. Immunogenicity of combined hepatitis A and B vaccine in elderly persons. Vaccine. 2003;21:3623–8. https://doi.org/10.1016/S0264-410X(03)00399-2 .
Fisman DN, Agrawal D, Leder K. The effect of age on immunologic response to recombinant hepatitis B vaccine: A meta-analysis. Clin Infect Dis. 2002;35:1368–75. https://doi.org/10.1086/344271 .
Stoffel M, Lievens M, Dieussaert I, Martin I, André F. Immunogenicity of Twinrix TM in older adults: A critical analysis. Expert Rev Vaccines. 2003;2:9–14. https://doi.org/10.1586/14760584.2.1.9 .
Weinberger B, Haks MC, de Paus RA, Ottenhoff THM, Bauer T, Grubeck-Loebenstein B. Impaired immune response to primary but not to booster vaccination against hepatitis B in older adults. Front Immunol. 2018. https://doi.org/10.3389/fimmu.2018.01035 .
Rafferty E, Duclos P, Yactayo S, Schuster M. Risk of yellow fever vaccine-associated viscerotropic disease among the elderly: a systematic review. Vaccine. 2013;31:5798–805. https://doi.org/10.1016/j.vaccine.2013.09.030 .
Poolman JT, Anderson AS. Escherichia coli and Staphylococcus aureus: leading bacterial pathogens of healthcare associated infections and bacteremia in older-age populations. Expert Rev Vaccines. 2018;17:607–18. https://doi.org/10.1080/14760584.2018.1488590 .
Esposito S, Principi N. Norovirus Vaccine: Priorities for Future Research and Development. Front Immunol. 2020;11:1383. https://doi.org/10.3389/fimmu.2020.01383 .
Anderson AS, Scully IL, Pride MW, Jansen KU. Vaccination against Nosocomial Infections in Elderly Adults. Interdiscip Top Gerontol Geriatr. 2020;43:193–217. https://doi.org/10.1159/000504481 .
Fleming DM, Taylor RJ, Lustig RL, Schuck-Paim C, Haguinet F, Webb DJ, et al. Modelling estimates of the burden of Respiratory Syncytial virus infection in adults and the elderly in the United Kingdom. BMC Infect Dis. 2015;15. https://doi.org/10.1186/s12879-015-1218-z .
Mazur NI, Higgins D, Nunes MC, Melero JA, Langedijk AC, Horsley N, et al. The respiratory syncytial virus vaccine landscape: lessons from the graveyard and promising candidates. Lancet Infect Dis. 2018;18:e295–311. https://doi.org/10.1016/S1473-3099(18)30292-5 .
Download references
Acknowledgements
Authors´ contributions.
BW wrote the manuscript. The author read and approved the final manuscript.
BW has received funding from the Innovative Medicines Initiative 2 Joint Undertaking (JU) under grant agreement No 806776. The JU receives support from the European Union’s Horizon 2020 research and innovation programme and EFPIA.
Author information
Authors and affiliations.
Institute for Biomedical Aging Research, Universität Innsbruck, Rennweg 10, 6020, Innsbruck, Austria
Birgit Weinberger
You can also search for this author in PubMed Google Scholar
Corresponding author
Correspondence to Birgit Weinberger .
Ethics declarations
Ethics approval and consent to participate, consent for publication, competing interests.
The author declares that she has no competing interests
Additional information
Publisher’s note.
Springer Nature remains neutral with regard to jurisdictional claims in published maps and institutional affiliations.
Rights and permissions
Open Access This article is licensed under a Creative Commons Attribution 4.0 International License, which permits use, sharing, adaptation, distribution and reproduction in any medium or format, as long as you give appropriate credit to the original author(s) and the source, provide a link to the Creative Commons licence, and indicate if changes were made. The images or other third party material in this article are included in the article's Creative Commons licence, unless indicated otherwise in a credit line to the material. If material is not included in the article's Creative Commons licence and your intended use is not permitted by statutory regulation or exceeds the permitted use, you will need to obtain permission directly from the copyright holder. To view a copy of this licence, visit http://creativecommons.org/licenses/by/4.0/ . The Creative Commons Public Domain Dedication waiver ( http://creativecommons.org/publicdomain/zero/1.0/ ) applies to the data made available in this article, unless otherwise stated in a credit line to the data.
Reprints and permissions
About this article
Cite this article.
Weinberger, B. Vaccination of older adults: Influenza, pneumococcal disease, herpes zoster, COVID-19 and beyond. Immun Ageing 18 , 38 (2021). https://doi.org/10.1186/s12979-021-00249-6
Download citation
Received : 24 August 2021
Accepted : 21 September 2021
Published : 09 October 2021
DOI : https://doi.org/10.1186/s12979-021-00249-6
Share this article
Anyone you share the following link with will be able to read this content:
Sorry, a shareable link is not currently available for this article.
Provided by the Springer Nature SharedIt content-sharing initiative
- Vaccination
- Streptococcus pneumoniae
Immunity & Ageing
ISSN: 1742-4933
- General enquiries: [email protected]
Volume 26, Number 5—May 2020
Policy Review
Nonpharmaceutical measures for pandemic influenza in nonhealthcare settings—personal protective and environmental measures.

Cite This Article
There were 3 influenza pandemics in the 20th century, and there has been 1 so far in the 21st century. Local, national, and international health authorities regularly update their plans for mitigating the next influenza pandemic in light of the latest available evidence on the effectiveness of various control measures in reducing transmission. Here, we review the evidence base on the effectiveness of nonpharmaceutical personal protective measures and environmental hygiene measures in nonhealthcare settings and discuss their potential inclusion in pandemic plans. Although mechanistic studies support the potential effect of hand hygiene or face masks, evidence from 14 randomized controlled trials of these measures did not support a substantial effect on transmission of laboratory-confirmed influenza. We similarly found limited evidence on the effectiveness of improved hygiene and environmental cleaning. We identified several major knowledge gaps requiring further research, most fundamentally an improved characterization of the modes of person-to-person transmission.
Influenza pandemics occur at irregular intervals when new strains of influenza A virus spread in humans ( 1 ). Influenza pandemics cause considerable health and social impact that exceeds that of typical seasonal (interpandemic) influenza epidemics. One of the characteristics of influenza pandemics is the high incidence of infections in all age groups because of the lack of population immunity. Although influenza vaccines are the cornerstone of seasonal influenza control, specific vaccines for a novel pandemic strain are not expected to be available for the first 5–6 months of the next pandemic. Antiviral drugs will be available in some locations to treat more severe infections but are unlikely to be available in the quantities that might be required to control transmission in the general community. Thus, efforts to control the next pandemic will rely largely on nonpharmaceutical interventions.
Most influenza virus infections cause mild and self-limiting disease; only a small fraction of case-patients require hospitalization. Therefore, influenza virus infections spread mainly in the community. Influenza virus is believed to be transmitted predominantly by respiratory droplets, but the size distribution of particles responsible for transmission remains unclear, and in particular, there is a lack of consensus on the role of fine particle aerosols in transmission ( 2 , 3 ). In healthcare settings, droplet precautions are recommended in addition to standard precautions for healthcare personnel when interacting with influenza patients and for all visitors during influenza seasons ( 4 ). Outside healthcare settings, hand hygiene is recommended in most national pandemic plans ( 5 ), and medical face masks were a common sight during the influenza pandemic in 2009. Hand hygiene has been proven to prevent many infectious diseases and might be considered a major component in influenza pandemic plans, whether or not it has proven effectiveness against influenza virus transmission, specifically because of its potential to reduce other infections and thereby reduce pressure on healthcare services.
In this article, we review the evidence base for personal protective measures and environmental hygiene measures, and specifically the evidence for the effectiveness of these measures in reducing transmission of laboratory-confirmed influenza in the community. We also discuss the implications of the evidence base for inclusion of these measures in pandemic plans.
Methods and Results
We conducted systematic reviews to evaluate the effectiveness of personal protective measures on influenza virus transmission, including hand hygiene, respiratory etiquette, and face masks, and a systematic review of surface and object cleaning as an environmental measure ( Table 1 ). We searched 4 databases (Medline, PubMed, EMBASE, and CENTRAL) for literature in all languages. We aimed to identify randomized controlled trials (RCTs) of each measure for laboratory-confirmed influenza outcomes for each of the measures because RCTs provide the highest quality of evidence. For respiratory etiquette and surface and object cleaning, because of a lack of RCTs for laboratory-confirmed influenza, we also searched for RCTs reporting effects of these interventions on influenza-like illness (ILI) and respiratory illness outcomes and then for observational studies on laboratory-confirmed influenza, ILI, and respiratory illness outcomes. For each review, 2 authors (E.Y.C.S. and J.X.) screened titles and abstracts and reviewed full texts independently.
We performed meta-analysis for hand hygiene and face mask interventions and estimated the effect of these measures on laboratory-confirmed influenza prevention by risk ratios (RRs). We used a fixed-effects model to estimate the overall effect in a pooled analysis or subgroup analysis. No overall effect would be generated if there was considerable heterogeneity on the basis of I 2 statistic > 75% ( 6 ). We performed quality assessment of evidence on hand hygiene and face mask interventions by using the GRADE (Grading of Recommendations Assessment, Development and Evaluation) approach ( 7 ). We provide additional details of the search strategies, selection of articles, summaries of the selected articles, and quality assessment ( Appendix ).
Personal Protective Measures
Hand hygiene.
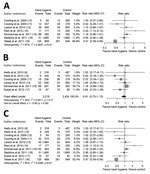
Figure 1 . Meta-analysis of risk ratios for the effect of hand hygiene with or without face mask use on laboratory-confirmed influenza from 10 randomized controlled trials with >11,000 participants. A) Hand hygiene alone;...
We identified a recent systematic review by Wong et al. on RCTs designed to assess the efficacy of hand hygiene interventions against transmission of laboratory-confirmed influenza ( 8 ). We used this review as a starting point and then searched for additional literature published after 2013; we found 3 additional eligible articles published during the search period of January 1, 2013–August 13, 2018. In total, we identified 12 articles ( 9 – 20 ), of which 3 articles were from the updated search and 9 articles from Wong et al. ( 8 ). Two articles relied on the same underlying dataset ( 16 , 19 ); therefore, we counted these 2 articles as 1 study, which resulted in 11 RCTs. We further selected 10 studies with >10,000 participants for inclusion in the meta-analysis ( Figure 1 ). We excluded 1 study from the meta-analysis because it provided estimates of infection risks only at the household level, not the individual level ( 20 ). We did not generate an overall pooled effect of hand hygiene only or of hand hygiene with or without face mask because of high heterogeneity in individual estimates ( I 2 87 and 82%, respectively). The effect of hand hygiene combined with face masks on laboratory-confirmed influenza was not statistically significant (RR 0.91, 95% CI 0.73–1.13; I 2 = 35%, p = 0.39). Some studies reported being underpowered because of limited sample size, and low adherence to hand hygiene interventions was observed in some studies.
We further analyzed the effect of hand hygiene by setting because transmission routes might vary in different settings. We found 6 studies in household settings examining the effect of hand hygiene with or without face masks, but the overall pooled effect was not statistically significant (RR 1.05, 95% CI 0.86–1.27; I 2 = 57%, p = 0.65) ( Appendix Figure 4) ( 11 – 15 , 17 ). The findings of 2 studies in school settings were different ( Appendix Figure 5). A study conducted in the United States ( 16 ) showed no major effect of hand hygiene, whereas a study in Egypt ( 18 ) reported that hand hygiene reduced the risk for influenza by >50%. A pooled analysis of 2 studies in university residential halls reported a marginally significant protective effect of a combination of hand hygiene plus face masks worn by all residents (RR 0.48, 95% CI 0.21–1.08; I 2 = 0%, p = 0.08) ( Appendix Figure 6) ( 9 , 10 ).
In support of hand hygiene as an effective measure, experimental studies have reported that influenza virus could survive on human hands for a short time and could transmit between hands and contaminated surfaces ( 2 , 21 ). Some field studies reported that influenza A(H1N1)pdm09 and influenza A(H3N2) virus RNA and viable influenza virus could be detected on the hands of persons with laboratory-confirmed influenza ( 22 , 23 ), supporting the potential of direct and indirect contact transmission to play a role in the spread of influenza. Other experimental studies also demonstrated that hand hygiene could reduce or remove infectious influenza virus from human hands ( 24 , 25 ). However, results from our meta-analysis on RCTs did not provide evidence to support a protective effect of hand hygiene against transmission of laboratory-confirmed influenza. One study did report a major effect, but in this trial of hand hygiene in schools in Egypt, running water had to be installed and soap and hand-drying material had to be introduced into the intervention schools as part of the project ( 18 ). Therefore, the impact of hand hygiene might also be a reflection of the introduction of soap and running water into primary schools in a lower-income setting. If one considers all of the evidence from RCTs together, it is useful to note that some studies might have underestimated the true effect of hand hygiene because of the complexity of implementing these intervention studies. For instance, the control group would not typically have zero knowledge or use of hand hygiene, and the intervention group might not adhere to optimal hand hygiene practices ( 11 , 13 , 15 ).
Hand hygiene is also effective in preventing other infectious diseases, including diarrheal diseases and some respiratory diseases ( 8 , 26 ). The need for hand hygiene in disease prevention is well recognized among most communities. Hand hygiene has been accepted as a personal protective measure in >50% of national preparedness plans for pandemic influenza ( 5 ). Hand hygiene practice is commonly performed with soap and water, alcohol-based hand rub, or other waterless hand disinfectants, all of which are easily accessible, available, affordable, and well accepted in most communities. However, resource limitations in some areas are a concern when clean running water or alcohol-based hand rub are not available. There are few adverse effects of hand hygiene except for skin irritation caused by some hand hygiene products ( 27 ). However, because of certain social or religious practices, alcohol-based hand sanitizers might not be permitted in some locations ( 28 ). Compliance with proper hand hygiene practice tends to be low because habitual behaviors are difficult to change ( 29 ). Therefore, hand hygiene promotion programs are needed to advocate and encourage proper and effective hand hygiene.
Respiratory Etiquette
Respiratory etiquette is defined as covering the nose and mouth with a tissue or a mask (but not a hand) when coughing or sneezing, followed by proper disposal of used tissues, and proper hand hygiene after contact with respiratory secretions ( 30 ). Other descriptions of this measure have included turning the head and covering the mouth when coughing and coughing or sneezing into a sleeve or elbow, rather than a hand. The rationale for not coughing into hands is to prevent subsequent contamination of other surfaces or objects ( 31 ). We conducted a search on November 6, 2018, and identified literature that was available in the databases during 1946–November 5, 2018. We did not identify any published research on the effectiveness of respiratory etiquette in reducing the risk for laboratory-confirmed influenza or ILI. One observational study reported a similar incidence rate of self-reported respiratory illness (defined by > 1 symptoms: cough, congestion, sore throat, sneezing, or breathing problems) among US pilgrims with or without practicing respiratory etiquette during the Hajj ( 32 ). The authors did not specify the type of respiratory etiquette used by participants in the study. A laboratory-based study reported that common respiratory etiquette, including covering the mouth by hands, tissue, or sleeve/arm, was fairly ineffective in blocking the release and dispersion of droplets into the surrounding environment on the basis of measurement of emitted droplets with a laser diffraction system ( 31 ).
Respiratory etiquette is often listed as a preventive measure for respiratory infections. However, there is a lack of scientific evidence to support this measure. Whether respiratory etiquette is an effective nonpharmaceutical intervention in preventing influenza virus transmission remains questionable, and worthy of further research.
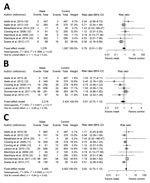
Figure 2 . Meta-analysis of risk ratios for the effect of face mask use with or without enhanced hand hygiene on laboratory-confirmed influenza from 10 randomized controlled trials with >6,500 participants. A) Face mask...
In our systematic review, we identified 10 RCTs that reported estimates of the effectiveness of face masks in reducing laboratory-confirmed influenza virus infections in the community from literature published during 1946–July 27, 2018. In pooled analysis, we found no significant reduction in influenza transmission with the use of face masks (RR 0.78, 95% CI 0.51–1.20; I 2 = 30%, p = 0.25) ( Figure 2 ). One study evaluated the use of masks among pilgrims from Australia during the Hajj pilgrimage and reported no major difference in the risk for laboratory-confirmed influenza virus infection in the control or mask group ( 33 ). Two studies in university settings assessed the effectiveness of face masks for primary protection by monitoring the incidence of laboratory-confirmed influenza among student hall residents for 5 months ( 9 , 10 ). The overall reduction in ILI or laboratory-confirmed influenza cases in the face mask group was not significant in either studies ( 9 , 10 ). Study designs in the 7 household studies were slightly different: 1 study provided face masks and P2 respirators for household contacts only ( 34 ), another study evaluated face mask use as a source control for infected persons only ( 35 ), and the remaining studies provided masks for the infected persons as well as their close contacts ( 11 – 13 , 15 , 17 ). None of the household studies reported a significant reduction in secondary laboratory-confirmed influenza virus infections in the face mask group ( 11 – 13 , 15 , 17 , 34 , 35 ). Most studies were underpowered because of limited sample size, and some studies also reported suboptimal adherence in the face mask group.
Disposable medical masks (also known as surgical masks) are loose-fitting devices that were designed to be worn by medical personnel to protect accidental contamination of patient wounds, and to protect the wearer against splashes or sprays of bodily fluids ( 36 ). There is limited evidence for their effectiveness in preventing influenza virus transmission either when worn by the infected person for source control or when worn by uninfected persons to reduce exposure. Our systematic review found no significant effect of face masks on transmission of laboratory-confirmed influenza.
We did not consider the use of respirators in the community. Respirators are tight-fitting masks that can protect the wearer from fine particles ( 37 ) and should provide better protection against influenza virus exposures when properly worn because of higher filtration efficiency. However, respirators, such as N95 and P2 masks, work best when they are fit-tested, and these masks will be in limited supply during the next pandemic. These specialist devices should be reserved for use in healthcare settings or in special subpopulations such as immunocompromised persons in the community, first responders, and those performing other critical community functions, as supplies permit.
In lower-income settings, it is more likely that reusable cloth masks will be used rather than disposable medical masks because of cost and availability ( 38 ). There are still few uncertainties in the practice of face mask use, such as who should wear the mask and how long it should be used for. In theory, transmission should be reduced the most if both infected members and other contacts wear masks, but compliance in uninfected close contacts could be a problem ( 12 , 34 ). Proper use of face masks is essential because improper use might increase the risk for transmission ( 39 ). Thus, education on the proper use and disposal of used face masks, including hand hygiene, is also needed.
Environmental Measures
Surface and object cleaning.
For the search period from 1946 through October 14, 2018, we identified 2 RCTs and 1 observational study about surface and object cleaning measures for inclusion in our systematic review ( 40 – 42 ). One RCT conducted in day care nurseries found that biweekly cleaning and disinfection of toys and linen reduced the detection of multiple viruses, including adenovirus, rhinovirus, and respiratory syncytial virus in the environment, but this intervention was not significant in reducing detection of influenza virus, and it had no major protective effect on acute respiratory illness ( 41 ). Another RCT found that hand hygiene with hand sanitizer together with surface disinfection reduced absenteeism related to gastrointestinal illness in elementary schools, but there was no major reduction in absenteeism related to respiratory illness ( 42 ). A cross-sectional study found that passive contact with bleach was associated with a major increase in self-reported influenza ( 40 ).
Given that influenza virus can survive on some surfaces for prolonged periods ( 43 ), and that cleaning or disinfection procedures can effectively reduce or inactivate influenza virus from surfaces and objects in experimental studies ( 44 ), there is a theoretical basis to believe that environmental cleaning could reduce influenza transmission. As an illustration of this proposal, a modeling study estimated that cleaning of extensively touched surfaces could reduce influenza A infection by 2% ( 45 ). However, most studies of influenza virus in the environment are based on detection of virus RNA by PCR, and few studies reported detection of viable virus.
Although we found no evidence that surface and object cleaning could reduce influenza transmission, this measure does have an established impact on prevention of other infectious diseases ( 42 ). It should be feasible to implement this measure in most settings, subject to the availability of water and cleaning products. Although irritation caused by cleaning products is limited, safety remains a concern because some cleaning products can be toxic or cause allergies ( 40 ).
In this review, we did not find evidence to support a protective effect of personal protective measures or environmental measures in reducing influenza transmission. Although these measures have mechanistic support based on our knowledge of how influenza is transmitted from person to person, randomized trials of hand hygiene and face masks have not demonstrated protection against laboratory-confirmed influenza, with 1 exception ( 18 ). We identified only 2 RCTs on environmental cleaning and no RCTs on cough etiquette.
Hand hygiene is a widely used intervention and has been shown to effectively reduce the transmission of gastrointestinal infections and respiratory infections ( 26 ). However, in our systematic review, updating the findings of Wong et al. ( 8 ), we did not find evidence of a major effect of hand hygiene on laboratory-confirmed influenza virus transmission ( Figure 1 ). Nevertheless, hand hygiene might be included in influenza pandemic plans as part of general hygiene and infection prevention.
We did not find evidence that surgical-type face masks are effective in reducing laboratory-confirmed influenza transmission, either when worn by infected persons (source control) or by persons in the general community to reduce their susceptibility ( Figure 2 ). However, as with hand hygiene, face masks might be able to reduce the transmission of other infections and therefore have value in an influenza pandemic when healthcare resources are stretched.
It is essential to note that the mechanisms of person-to-person transmission in the community have not been fully determined. Controversy remains over the role of transmission through fine-particle aerosols ( 3 , 46 ). Transmission by indirect contact requires transfer of viable virus from respiratory mucosa onto hands and other surfaces, survival on those surfaces, and successful inoculation into the respiratory mucosa of another person. All of these components of the transmission route have not been studied extensively. The impact of environmental factors, such as temperature and humidity, on influenza transmission is also uncertain ( 47 ). These uncertainties over basic transmission modes and mechanisms hinder the optimization of control measures.
In this review, we focused on 3 personal protective measures and 1 environmental measure. Other potential environmental measures include humidification in dry environments ( 48 ), increasing ventilation ( 49 ), and use of upper-room UV light ( 50 ), but there is limited evidence to support these measures. Further investigations on the effectiveness of respiratory etiquette and surface cleaning through conducting RCTs would be helpful to provide evidence with higher quality; evaluation of the effectiveness of these measures targeting specific population groups, such as immunocompromised persons, would also be beneficial ( Table 2 ). Future cost-effectiveness evaluations could provide more support for the potential use of these measures. Further research on transmission modes and alternative interventions to reduce influenza transmission would be valuable in improving pandemic preparedness. Finally, although our review focused on nonpharmaceutical measures to be taken during influenza pandemics, the findings could also apply to severe seasonal influenza epidemics. Evidence from RCTs of hand hygiene or face masks did not support a substantial effect on transmission of laboratory-confirmed influenza, and limited evidence was available on other environmental measures.
Ms. Xiao is a postgraduate student at the School of Public Health, University of Hong Kong, Hong Kong, China. Her primary research interests are influenza epidemiology and the dynamics of person-to-person transmission.
Acknowledgments
This study was conducted in preparation for the development of guidelines by the World Health Organization on the use of nonpharmaceutical interventions for pandemic influenza in nonmedical settings.
This study was supported by the World Health Organization. J.X. and M.W.F. were supported by the Collaborative Research Fund from the University Grants Committee of Hong Kong (project no. C7025-16G).
- Uyeki TM , Katz JM , Jernigan DB . Novel influenza A viruses and pandemic threats. Lancet . 2017 ; 389 : 2172 – 4 . DOI PubMed Google Scholar
- Bean B , Moore BM , Sterner B , Peterson LR , Gerding DN , Balfour HH Jr . Survival of influenza viruses on environmental surfaces. J Infect Dis . 1982 ; 146 : 47 – 51 . DOI PubMed Google Scholar
- Tellier R . Aerosol transmission of influenza A virus: a review of new studies. J R Soc Interface . 2009 ; 6 ( Suppl 6 ): S783 – 90 . DOI PubMed Google Scholar
- Siegel JD , Rhinehart E , Jackson M , Chiarello L ; Health Care Infection Control Practices Advisory Committee . 2007 guideline for isolation precautions: preventing transmission of infectious agents in health care settings: Atlanta: Centers for Disease Control and Prevention; 2007 .
- World Health Organization . Comparative analysis of national pandemic influenza preparedness plans, 2011 [cited 2019 Jun 25]. https://www.who.int/influenza/resources/documents/comparative_analysis_php_2011_en.pdf
- Guyatt GH , Oxman AD , Kunz R , Woodcock J , Brozek J , Helfand M , et al. ; GRADE Working Group . GRADE guidelines: 7. Rating the quality of evidence—inconsistency. J Clin Epidemiol . 2011 ; 64 : 1294 – 302 . DOI PubMed Google Scholar
- Guyatt G , Oxman AD , Akl EA , Kunz R , Vist G , Brozek J , et al. GRADE guidelines: 1. Introduction-GRADE evidence profiles and summary of findings tables. J Clin Epidemiol . 2011 ; 64 : 383 – 94 . DOI PubMed Google Scholar
- Wong VW , Cowling BJ , Aiello AE . Hand hygiene and risk of influenza virus infections in the community: a systematic review and meta-analysis. Epidemiol Infect . 2014 ; 142 : 922 – 32 . DOI PubMed Google Scholar
- Aiello AE , Murray GF , Perez V , Coulborn RM , Davis BM , Uddin M , et al. Mask use, hand hygiene, and seasonal influenza-like illness among young adults: a randomized intervention trial. J Infect Dis . 2010 ; 201 : 491 – 8 . DOI PubMed Google Scholar
- Aiello AE , Perez V , Coulborn RM , Davis BM , Uddin M , Monto AS . Facemasks, hand hygiene, and influenza among young adults: a randomized intervention trial. PLoS One . 2012 ; 7 : e29744 . DOI PubMed Google Scholar
- Cowling BJ , Chan KH , Fang VJ , Cheng CK , Fung RO , Wai W , et al. Facemasks and hand hygiene to prevent influenza transmission in households: a cluster randomized trial. Ann Intern Med . 2009 ; 151 : 437 – 46 . DOI PubMed Google Scholar
- Cowling BJ , Fung RO , Cheng CK , Fang VJ , Chan KH , Seto WH , et al. Preliminary findings of a randomized trial of non-pharmaceutical interventions to prevent influenza transmission in households. PLoS One . 2008 ; 3 : e2101 . DOI PubMed Google Scholar
- Larson EL , Ferng YH , Wong-McLoughlin J , Wang S , Haber M , Morse SS . Impact of non-pharmaceutical interventions on URIs and influenza in crowded, urban households. Public Health Rep . 2010 ; 125 : 178 – 91 . DOI PubMed Google Scholar
- Ram PK , DiVita MA , Khatun-e-Jannat K , Islam M , Krytus K , Cercone E , et al. Impact of intensive handwashing promotion on secondary household influenza-like illness in rural bangladesh: findings from a randomized controlled trial. PLoS One . 2015 ; 10 : e0125200 . DOI PubMed Google Scholar
- Simmerman JM , Suntarattiwong P , Levy J , Jarman RG , Kaewchana S , Gibbons RV , et al. Findings from a household randomized controlled trial of hand washing and face masks to reduce influenza transmission in Bangkok, Thailand. Influenza Other Respir Viruses . 2011 ; 5 : 256 – 67 . DOI PubMed Google Scholar
- Stebbins S , Cummings DA , Stark JH , Vukotich C , Mitruka K , Thompson W , et al. Reduction in the incidence of influenza A but not influenza B associated with use of hand sanitizer and cough hygiene in schools: a randomized controlled trial. Pediatr Infect Dis J . 2011 ; 30 : 921 – 6 . DOI PubMed Google Scholar
- Suess T , Remschmidt C , Schink SB , Schweiger B , Nitsche A , Schroeder K , et al. The role of facemasks and hand hygiene in the prevention of influenza transmission in households: results from a cluster randomised trial; Berlin, Germany, 2009-2011. BMC Infect Dis . 2012 ; 12 : 26 . DOI PubMed Google Scholar
- Talaat M , Afifi S , Dueger E , El-Ashry N , Marfin A , Kandeel A , et al. Effects of hand hygiene campaigns on incidence of laboratory-confirmed influenza and absenteeism in schoolchildren, Cairo, Egypt. Emerg Infect Dis . 2011 ; 17 : 619 – 25 . DOI PubMed Google Scholar
- Azman AS , Stark JH , Althouse BM , Vukotich CJ Jr , Stebbins S , Burke DS , et al. Household transmission of influenza A and B in a school-based study of non-pharmaceutical interventions. Epidemics . 2013 ; 5 : 181 – 6 . DOI PubMed Google Scholar
- Levy JW , Suntarattiwong P , Simmerman JM , Jarman RG , Johnson K , Olsen SJ , et al. Increased hand washing reduces influenza virus surface contamination in Bangkok households, 2009-2010. Influenza Other Respir Viruses . 2014 ; 8 : 13 – 6 . DOI PubMed Google Scholar
- Mukherjee DV , Cohen B , Bovino ME , Desai S , Whittier S , Larson EL . Survival of influenza virus on hands and fomites in community and laboratory settings. Am J Infect Control . 2012 ; 40 : 590 – 4 . DOI PubMed Google Scholar
- Macias AE , de la Torre A , Moreno-Espinosa S , Leal PE , Bourlon MT , Ruiz-Palacios GM . Controlling the novel A (H1N1) influenza virus: don’t touch your face! J Hosp Infect . 2009 ; 73 : 280 – 1 . DOI PubMed Google Scholar
- Simmerman JM , Suntarattiwong P , Levy J , Gibbons RV , Cruz C , Shaman J , et al. Influenza virus contamination of common household surfaces during the 2009 influenza A (H1N1) pandemic in Bangkok, Thailand: implications for contact transmission. Clin Infect Dis . 2010 ; 51 : 1053 – 61 . DOI PubMed Google Scholar
- Grayson ML , Melvani S , Druce J , Barr IG , Ballard SA , Johnson PD , et al. Efficacy of soap and water and alcohol-based hand-rub preparations against live H1N1 influenza virus on the hands of human volunteers. Clin Infect Dis . 2009 ; 48 : 285 – 91 . DOI PubMed Google Scholar
- Larson EL , Cohen B , Baxter KA . Analysis of alcohol-based hand sanitizer delivery systems: efficacy of foam, gel, and wipes against influenza A (H1N1) virus on hands. Am J Infect Control . 2012 ; 40 : 806 – 9 . DOI PubMed Google Scholar
- Aiello AE , Coulborn RM , Perez V , Larson EL . Effect of hand hygiene on infectious disease risk in the community setting: a meta-analysis. Am J Public Health . 2008 ; 98 : 1372 – 81 . DOI PubMed Google Scholar
- Löffler H , Kampf G . Hand disinfection: how irritant are alcohols? J Hosp Infect . 2008 ; 70 ( Suppl 1 ): 44 – 8 . DOI PubMed Google Scholar
- Ahmed QA , Memish ZA , Allegranzi B , Pittet D , Global Patient Safety Challenge WHO ; WHO Global Patient Safety Challenge . Muslim health-care workers and alcohol-based handrubs. Lancet . 2006 ; 367 : 1025 – 7 . DOI PubMed Google Scholar
- Pittet D . Improving adherence to hand hygiene practice: a multidisciplinary approach. Emerg Infect Dis . 2001 ; 7 : 234 – 40 . DOI PubMed Google Scholar
- Centers for Disease Control and Prevention . Respiratory hygiene/cough etiquette in healthcare settings, 2009 [cited 2019 Jul 8]. https://www.cdc.gov/flu/professionals/infectioncontrol/resphygiene.htm
- Zayas G , Chiang MC , Wong E , MacDonald F , Lange CF , Senthilselvan A , et al. Effectiveness of cough etiquette maneuvers in disrupting the chain of transmission of infectious respiratory diseases. BMC Public Health . 2013 ; 13 : 811 . DOI PubMed Google Scholar
- Balaban V , Stauffer WM , Hammad A , Afgarshe M , Abd-Alla M , Ahmed Q , et al. Protective practices and respiratory illness among US travelers to the 2009 Hajj. J Travel Med . 2012 ; 19 : 163 – 8 . DOI PubMed Google Scholar
- Barasheed O , Almasri N , Badahdah AM , Heron L , Taylor J , McPhee K , et al. ; Hajj Research Team . Pilot randomised controlled trial to test effectiveness of facemasks in preventing influenza-like illness transmission among Australian Hajj pilgrims in 2011. Infect Disord Drug Targets . 2014 ; 14 : 110 – 6 . DOI PubMed Google Scholar
- MacIntyre CR , Cauchemez S , Dwyer DE , Seale H , Cheung P , Browne G , et al. Face mask use and control of respiratory virus transmission in households. Emerg Infect Dis . 2009 ; 15 : 233 – 41 . DOI PubMed Google Scholar
- MacIntyre CR , Zhang Y , Chughtai AA , Seale H , Zhang D , Chu Y , et al. Cluster randomised controlled trial to examine medical mask use as source control for people with respiratory illness. BMJ Open . 2016 ; 6 : e012330 . DOI PubMed Google Scholar
- US Food and Drug Administration . Masks and N95 respirators, 2018 [cited 2019 Jul 10]. https://www.fda.gov/medicaldevices/productsandmedicalprocedures/generalhospitaldevicesandsupplies/personalprotectiveequipment/ucm055977.htm
- Centers for Disease Control and Prevention . Respirator fact sheet, 2012 [cited 2019 Jul 10]. https://www.cdc.gov/niosh/npptl/topics/respirators/factsheets/respsars.html
- Chughtai AA , Seale H , MacIntyre CR . Use of cloth masks in the practice of infection control—evidence and policy gaps. Int J Infect Control. 2013 ; 9 : 1 – 12 . DOI Google Scholar
- World Health Organization . Advice on the use of masks in the community setting in Influenza A (H1N1) outbreaks, 2009 [cited 2019 Jul 10]. http://www.who.int/csr/resources/publications/Adviceusemaskscommunityrevised.pdf
- Casas L , Espinosa A , Borràs-Santos A , Jacobs J , Krop E , Heederik D , et al. Domestic use of bleach and infections in children: a multicentre cross-sectional study. Occup Environ Med . 2015 ; 72 : 602 – 4 . DOI PubMed Google Scholar
- Ibfelt T , Engelund EH , Schultz AC , Andersen LP . Effect of cleaning and disinfection of toys on infectious diseases and micro-organisms in daycare nurseries. J Hosp Infect . 2015 ; 89 : 109 – 15 . DOI PubMed Google Scholar
- Sandora TJ , Shih MC , Goldmann DA . Reducing absenteeism from gastrointestinal and respiratory illness in elementary school students: a randomized, controlled trial of an infection-control intervention. Pediatrics . 2008 ; 121 : e1555 – 62 . DOI PubMed Google Scholar
- Oxford J , Berezin EN , Courvalin P , Dwyer DE , Exner M , Jana LA , et al. The survival of influenza A(H1N1)pdm09 virus on 4 household surfaces. Am J Infect Control . 2014 ; 42 : 423 – 5 . DOI PubMed Google Scholar
- Tuladhar E , Hazeleger WC , Koopmans M , Zwietering MH , Beumer RR , Duizer E . Residual viral and bacterial contamination of surfaces after cleaning and disinfection. Appl Environ Microbiol . 2012 ; 78 : 7769 – 75 . DOI PubMed Google Scholar
- Zhang N , Li Y . Transmission of influenza A in a student office based on realistic person-to-person contact and surface touch behaviour. Int J Environ Res Public Health . 2018 ; 15 : E1699 . DOI PubMed Google Scholar
- Shiu EYC , Leung NHL , Cowling BJ . Controversy around airborne versus droplet transmission of respiratory viruses: implication for infection prevention. Curr Opin Infect Dis . 2019 ; 32 : 372 – 9 . DOI PubMed Google Scholar
- Marr LC , Tang JW , Van Mullekom J , Lakdawala SS . Mechanistic insights into the effect of humidity on airborne influenza virus survival, transmission and incidence. J R Soc Interface . 2019 ; 16 : 20180298 . DOI PubMed Google Scholar
- Reiman JM , Das B , Sindberg GM , Urban MD , Hammerlund MEM , Lee HB , et al. Humidity as a non-pharmaceutical intervention for influenza A. PLoS One . 2018 ; 13 : e0204337 . DOI PubMed Google Scholar
- Gao X , Wei J , Cowling BJ , Li Y . Potential impact of a ventilation intervention for influenza in the context of a dense indoor contact network in Hong Kong. Sci Total Environ . 2016 ; 569-570 : 373 – 81 . DOI PubMed Google Scholar
- McDevitt JJ , Rudnick SN , Radonovich LJ . Aerosol susceptibility of influenza virus to UV-C light. Appl Environ Microbiol . 2012 ; 78 : 1666 – 9 . DOI PubMed Google Scholar
- Figure 1 . Meta-analysis of risk ratios for the effect of hand hygiene with or without face mask use on laboratory-confirmed influenza from 10 randomized controlled trials with >11,000 participants. A) Hand...
- Figure 2 . Meta-analysis of risk ratios for the effect of face mask use with or without enhanced hand hygiene on laboratory-confirmed influenza from 10 randomized controlled trials with >6,500 participants. A)...
- Table 1 . Summary of literature searches for systematic review on personal and environmental nonpharmaceutical interventions for pandemic influenza
- Table 2 . Knowledge gaps for personal protective and environmental nonpharmaceutical interventions for pandemic influenza
DOI: 10.3201/eid2605.190994
Original Publication Date: February 06, 2020
1 These first authors contributed equally to this article.
Table of Contents – Volume 26, Number 5—May 2020
Please use the form below to submit correspondence to the authors or contact them at the following address:
Benjamin J. Cowling, World Health Organization Collaborating Centre for Infectious Disease Epidemiology and Control, School of Public Health, Li Ka Shing Faculty of Medicine, University of Hong Kong, 1/F Patrick Manson Bldg (North Wing), 7 Sassoon Rd, Hong Kong, China
Comment submitted successfully, thank you for your feedback.
There was an unexpected error. Message not sent.
Exit Notification / Disclaimer Policy
- The Centers for Disease Control and Prevention (CDC) cannot attest to the accuracy of a non-federal website.
- Linking to a non-federal website does not constitute an endorsement by CDC or any of its employees of the sponsors or the information and products presented on the website.
- You will be subject to the destination website's privacy policy when you follow the link.
- CDC is not responsible for Section 508 compliance (accessibility) on other federal or private website.
Article Citations
Highlight and copy the desired format.
Metric Details
Article views: 1695995.
Data is collected weekly and does not include downloads and attachments. View data is from .
Citations: 145
What is the altmetric attention score.
The Altmetric Attention Score for a research output provides an indicator of the amount of attention that it has received. The score is derived from an automated algorithm, and represents a weighted count of the amount of attention Altmetric picked up for a research output.
Influenza Epidemic Trend Surveillance and Prediction Based on Search Engine Data: Deep Learning Model Study
Affiliations.
- 1 Department of Management Science and Information System, Faculty of Management and Economics, Kunming University of Science and Technology, Kunming, China.
- 2 School of Population Medicine and Public Health, Chinese Academy of Medical Sciences & Peking Union Medical College, Beijing, China.
- 3 Department of Respiratory and Critical Care Medicine, Affiliated Hospital of Guilin Medical University, Guilin, China.
- 4 Department of Respiratory and Critical Care Medicine, Bejing Hospital, Beijing, China.
- 5 WorldPop, School of Geography and Environmental Science, University of Southampton, Southampton, United Kingdom.
- 6 The First People's Hospital of Yunnan Province, Affiliated Hospital of Kunming University of Science and Technology, Kunming, China.
- PMID: 37847532
- PMCID: PMC10618884
- DOI: 10.2196/45085
Background: Influenza outbreaks pose a significant threat to global public health. Traditional surveillance systems and simple algorithms often struggle to predict influenza outbreaks in an accurate and timely manner. Big data and modern technology have offered new modalities for disease surveillance and prediction. Influenza-like illness can serve as a valuable surveillance tool for emerging respiratory infectious diseases like influenza and COVID-19, especially when reported case data may not fully reflect the actual epidemic curve.
Objective: This study aimed to develop a predictive model for influenza outbreaks by combining Baidu search query data with traditional virological surveillance data. The goal was to improve early detection and preparedness for influenza outbreaks in both northern and southern China, providing evidence for supplementing modern intelligence epidemic surveillance methods.
Methods: We collected virological data from the National Influenza Surveillance Network and Baidu search query data from January 2011 to July 2018, totaling 3,691,865 and 1,563,361 respective samples. Relevant search terms related to influenza were identified and analyzed for their correlation with influenza-positive rates using Pearson correlation analysis. A distributed lag nonlinear model was used to assess the lag correlation of the search terms with influenza activity. Subsequently, a predictive model based on the gated recurrent unit and multiple attention mechanisms was developed to forecast the influenza-positive trend.
Results: This study revealed a high correlation between specific Baidu search terms and influenza-positive rates in both northern and southern China, except for 1 term. The search terms were categorized into 4 groups: essential facts on influenza, influenza symptoms, influenza treatment and medicine, and influenza prevention, all of which showed correlation with the influenza-positive rate. The influenza prevention and influenza symptom groups had a lag correlation of 1.4-3.2 and 5.0-8.0 days, respectively. The Baidu search terms could help predict the influenza-positive rate 14-22 days in advance in southern China but interfered with influenza surveillance in northern China.
Conclusions: Complementing traditional disease surveillance systems with information from web-based data sources can aid in detecting warning signs of influenza outbreaks earlier. However, supplementation of modern surveillance with search engine information should be approached cautiously. This approach provides valuable insights for digital epidemiology and has the potential for broader application in respiratory infectious disease surveillance. Further research should explore the optimization and customization of search terms for different regions and languages to improve the accuracy of influenza prediction models.
Keywords: early warning; epidemic intelligence; infectious disease; influenza-like illness; surveillance.
©Liuyang Yang, Ting Zhang, Xuan Han, Jiao Yang, Yanxia Sun, Libing Ma, Jialong Chen, Yanming Li, Shengjie Lai, Wei Li, Luzhao Feng, Weizhong Yang. Originally published in the Journal of Medical Internet Research (https://www.jmir.org), 17.10.2023.
Publication types
- Research Support, Non-U.S. Gov't
- COVID-19* / epidemiology
- China / epidemiology
- Deep Learning*
- Disease Outbreaks
- Influenza, Human* / epidemiology
- Search Engine
CDC Reports First U.S. Human Infection in 2024 with Variant Influenza Virus
April 5, 2024 —On March 29, 2024, CDC reported this year’s first U.S. human infection with an influenza (flu) virus that normally spreads in pigs and not people. The infection with an influenza A(H1N2) variant (v) virus occurred in a child living near a pig farm in Pennsylvania who had direct contact with pigs prior to illness onset. The person was hospitalized and has since recovered from their illness. CDC recommends that people in contact with pigs take precautions and provides specific guidance for people who are at higher risk of developing serious complications from flu.
The patient sought health care during the week ending March 9, 2024, and had the following symptoms: fever, vomiting, cough, and rhinorrhea (runny nose). The hospitalized patient received influenza antiviral treatment and recovered. The case was first reported by the Pennsylvania Department of Health. Local public health officials found that the patient had swine contact prior to illness onset. No person-to-person spread of the A(H1N2)v virus associated with this patient has been identified.
Additional investigation determined that two of the patient’s close contacts had also contact with swine and had been ill before the patient developed symptoms. The two persons had mild illness; their symptoms resolved, and they were not tested for influenza.
Variant influenza virus infections usually cause mild illness; however, they can cause severe illness and are concerning because of their pandemic potential. Similar to seasonal flu, people with certain underlying conditions are at higher risk of developing serious complications from infections with swine-origin influenza viruses.
First case of A(H1N2)v virus in the US in 2024
Every year, there are rare sporadic human infections with flu viruses that usually circulate in pigs. The first such infection with each novel influenza virus each year triggers international notification to global health authorities through International Health Regulations .
When a virus that normally spreads in pigs is found in people, these are called “variant flu virus” infections and are designated with the letter “v” after the subtype. Variant influenza A virus infections are novel influenza A virus infections. A novel influenza A virus is an influenza A virus that is different from seasonal influenza A viruses spreading among people.
Most human infections with variant influenza viruses occur following exposure to swine, but human-to-human spread can occur. However, in most cases, variant influenza viruses have not shown the ability to spread easily and sustainably from person to person. Variant influenza virus infections are usually associated with contact with pigs, often at agricultural fairs.
These infections are fully investigated to ensure that such viruses are not spreading efficiently in people and to limit further exposure of people to infected animals if infected animals are identified.
How Variant infections occur
Human infections with variant influenza viruses happen mainly when an infected pig coughs or sneezes, and droplets containing influenza virus spread through the air. If these droplets land in your nose or mouth or are inhaled, you can be infected.
There is also some evidence that you might get infected by touching something that has a virus on it and then touching your own eyes, mouth, or nose. CDC has information about how variant virus infections happen.
The number of variant virus infections in the United States has ranged from a high of 321 during 2011-2012 to a low of one during 2018-2019 and 2019-2020.
CDC Recommendations and Guidance
Besides recommendations for people in contact with pigs or people who are at higher risk of serious flu complications , as well as recommendations for fair exhibitors , CDC has web resources and background to understand swine flu infections better. CDC also has information on prevention and treatment for people with exposure to pigs.
Descargo de responsabilidad: Es posible que en este sitio encuentre algunos enlaces que le lleven a contenido disponible sólo en inglés. Además, el contenido que se ha traducido del inglés se actualiza a menudo , lo cual puede causar la aparición temporal de algunas partes en ese idioma hasta que se termine de traducir (generalmente en 24 horas). Llame al 1-800-CDC-INFO si tiene preguntas sobre la influenza estacional, cuyas respuestas no ha encontrado en este sitio. Agradecemos su paciencia.
- Communications Resource Center
- International Work
- Outbreak Investigations
To receive weekly email updates about Seasonal Flu, enter your email address:
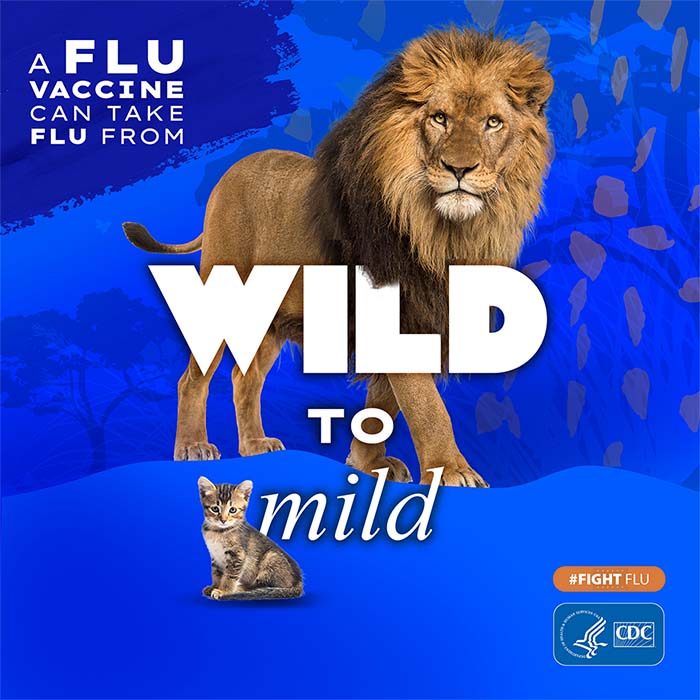
- Influenza in Animals
Exit Notification / Disclaimer Policy
- The Centers for Disease Control and Prevention (CDC) cannot attest to the accuracy of a non-federal website.
- Linking to a non-federal website does not constitute an endorsement by CDC or any of its employees of the sponsors or the information and products presented on the website.
- You will be subject to the destination website's privacy policy when you follow the link.
- CDC is not responsible for Section 508 compliance (accessibility) on other federal or private website.

An official website of the United States government
The .gov means it’s official. Federal government websites often end in .gov or .mil. Before sharing sensitive information, make sure you’re on a federal government site.
The site is secure. The https:// ensures that you are connecting to the official website and that any information you provide is encrypted and transmitted securely.
- Publications
- Account settings
Preview improvements coming to the PMC website in October 2024. Learn More or Try it out now .
- Advanced Search
- Journal List
- J Gen Virol

The first decade of research advances in influenza D virus
1 Department of Veterinary Science, Maxwell H. Gluck Equine Research Center, University of Kentucky, Lexington, Kentucky 40546, USA
From its initial isolation in the USA in 2011 to the present, influenza D virus (IDV) has been detected in cattle and swine populations worldwide. IDV has exceptional thermal and acid stability and a broad host range. The virus utilizes cattle as its natural reservoir and amplification host with periodic spillover to other mammalian species, including swine. IDV infection can cause mild to moderate respiratory illnesses in cattle and has been implicated as a contributor to bovine respiratory disease (BRD) complex, which is the most common and costly disease affecting the cattle industry. Bovine and swine IDV outbreaks continue to increase globally, and there is increasing evidence indicating that IDV may have the potential to infect humans. This review discusses recent advances in IDV biology and epidemiology, and summarizes our current understanding of IDV pathogenesis and zoonotic potential.
Introduction
Influenza viruses are enveloped, segmented, single-stranded, negative-sense RNA viruses that belong to the family Orthomyxoviridae . Four influenza genera, Alphainfluenzavirus (species: Influenza A virus ), Betainfluenzavirus (species: Influenza B virus ), Gammainfluenzavirus (species: Influenza C virus ) and Deltainfluenzavirus (species: Influenza D virus ) [ 1 ], are classified on the basis of antigenic differences in the nucleocapsid protein (NP) and matrix protein (M). Influenza A virus (IAV) can be further divided into subtypes based on the haemagglutinin (HA) and neuraminidase (NA) glycoproteins. To date, 18 different HA subtypes (H1–H18) and 11 different NA subtypes (N1–N11) have been identified in IAV. H1N1 and H3N2 of IAV are currently endemic in the global human population [ 2 ]. Influenza B virus (IBV), according to the major surface HA antigen, can be further broken down into two lineages (B/Yamagata and B/Victoria) [ 1 ]. Influenza C virus (ICV) has six evolutionary lineages represented by Taylor/47, Kanagawa/1/76, Yamagata/26/81, Aichi/1/81, Sao Paulo/378/82 and Mississippi/80 [ 3 ]. Influenza D virus (IDV) was first isolated in 2011 [ 4 ] and officially named in 2016 ( https://www.cdc.gov/flu/about/viruses/types.htm ). IDV can be genetically classified into four lineages: D/OK, D/660, D/Yama2016 and D/Yama2019, based on the hemagglutinin-esterase-fusion (HEF) gene [ 5, 6 ], which is the primary target of neutralizing antibodies generated during IDV infection.
The influenza A and B virus genomes each consist of eight segments, while influenza C and D virus genomes each contain seven segments and lack a neuraminidase (NA) segment ( Fig. 1a ). The HEF segment of ICV and IDV encodes a major envelope glycoprotein that mediates the receptor binding, membrane fusion and esterase activity as compared to the functions in both HA and NA proteins of IAV and IBV. Among the four types of influenza viruses ( Fig. 1b ), IAV is the most common and the most pathogenic, causing seasonal epidemics every year and, on some occasions, pandemics. IAV infects a wide variety of hosts, including humans, birds, horses, pigs, dogs and marine mammals [ 2, 7 ]. Waterfowl are the major reservoir of IAV, harbouring all different subtypes of viruses, with the potential for reassortment, producing new antigenic variants to which humans do not have pre-existing immunity [ 8 ]. Bird-origin influenza viruses can cross the species barrier and infect humans to cause pandemics. There were three recorded pandemics in the last century, including the 1918 H1N1 Spanish pandemic, the 1957 H2N2 Asian pandemic and the 1968 H3N2 Hong Kong pandemic [ 9 ]. In 2009, a novel swine-origin H1N1 influenza virus emerged to cause the first human influenza pandemic of the 21st century [ 2 ]. Various other influenza A viruses that originated from swine and birds, such as H5, H6, H7, H9 and H10, also have the capacity to infect and spread among humans and, in some cases, result in severe disease and death [ 2, 9 ]. IBV, like IAV, can cause seasonal epidemics in humans but lacks the ability to trigger influenza pandemics ( Fig. 1b ). Approximately 25 % of the clinical cases in seasonal influenza are caused by IBV infection, and sometimes IBV can dominate influenza seasons, as in the 2017–2018 seasonal influenza in Europe [ 10 ]. IBV utilizes humans as its primary reservoir [ 11 ]. Although there were cases of IBV crossing over from humans to other species, such as the IBV found in seals or pigs, IBV is largely restricted to circulation in humans [ 11 ]. ICV utilizes humans as a reservoir and is thought to cause mild respiratory symptoms in humans [ 12 ] ( Fig. 1b ). Some studies have demonstrated that ICV has the ability to infect and replicate in pigs, dogs and bovines [ 13 ]. The presence of ICV in animals may suggest its spillover events from the human reservoir. Periodic epidemics of ICV infection have also been reported [ 14, 15 ].

Overview of four types of influenza viruses. (a) Genomes and viral particles of influenza A, B, C and D viruses. Influenza A and B virus particles possess eight genomic segments (left), while influenza C and D virus particles contain seven genomic segments (right). The envelope and membrane proteins HEF (ICV/IDV), HA and NA (IAV/IBV), and M2 project from the virion surface with the M1 protein just underneath the inner leaflet of the membrane, which encases the virion core containing the virus genome. Each vRNA segment forms a vRNP together with the NP and RNA polymerase complex (PB2, PB1 and PA/P3). (b) Summary of general characteristics of four types of influenza viruses.
IDV uses cattle as its primary reservoir and can be transmitted from cattle to swine [ 4, 16, 17 ] ( Fig. 1b ). Antibodies specific for IDV were also detected in horses, small ruminants, feral swine, buffalo, camels and humans, especially those exposed to cattle [ 18–21 ], indicating that IDV may have a wide host range and can spread from cattle to humans or other animal species. In this review, recent advances in influenza D virus research are summarized, and this is followed by discussion of how novel knowledge about IDV discriminates it from other types of influenza viruses, especially the closely related influenza C virus.
Epidemiology of IDV worldwide
Idv in north america.
In April 2011, a viral isolate with approximately 50 % amino acid identity to the human ICV was identified from a clinically ill pig with influenza-like symptoms in Oklahoma, USA [ 4 ]. The virus was initially thought to be a new subtype of ICV. However, further characterization provided evidence that it was genetically, antigenically and biologically distinct from human ICV [ 16 ]. In August 2016, the International Committee on Taxonomy of Viruses (ICTV) classified this novel virus as a new genus ( Deltainfluenzavirus, species: Influenza D virus ) of the family Orthomyxoviridae .
Although the first IDV was isolated from a diseased pig, subsequent follow-up studies demonstrated that IDV is widespread in cattle [ 16, 22 ]. Agricultural cattle had not been considered to be susceptible to influenza viruses until the discovery of IDV [ 23 ]. Initial epidemiological investigation of IDV in pigs showed a low seropositive rate (9.5 %, n =220) ( Table 1 ) [ 4 ]. In addition, the IDV positivity percentage was <0.1 % as determined by RT-PCR during routine testing of nasal swabs from pigs, and the isolation of IDV from pigs was also infrequent [ 16 ]. In contrast, a higher positivity rate (18 %, n =48) for IDV was found in diagnostic testing of nasal swabs from cattle with respiratory symptoms using RT-PCR ( Table 1 ), and viruses were successfully isolated from five of the eight positive samples [ 16 ]. A surveillance study also found that 10 of the 208 clinical samples (4.8 %) submitted for laboratory diagnosis of bovine respiratory disease (BRD) complex tested positive for IDV using RT-PCR ( Table 1 ), and 6 viruses were isolated from the 10 IDV-positive samples [ 22 ]. Moreover, two published studies in young calves reported that 94 and 98 % of neonatal cattle acquired high levels of maternal antibodies against IDV, respectively [ 24, 25 ]. These serological studies also demonstrated that IDV had been present in US cattle herds (Mississippi and Nebraska) since as early as 2003 [ 24, 25 ]. A nationwide serosurveillance for IDV in the USA found an overall seropositivity rate of 77.5 % ( n =1992) in cattle serum samples collected across the country in 2014–1015 ( Table 1 ), but the prevalence rates varied from 47.7–84.6 % among different regions [ 26 ]. Furthermore, seropositive samples were found in 41 of the 42 states in the USA, indicating wide circulation of IDV in the US cattle population during 2014–2015 [ 26 ]. Two studies conducted using metagenomic sequencing of samples collected from feedlot cattle in the USA, Canada and Mexico demonstrated that IDV was significantly associated with BRD [ 27, 28 ]. The widespread presence of IDV and the relative ease of the virus isolation in cattle suggested that cattle represent a major replication host and a reservoir for IDV [ 16 ].
The epidemiology of IDV worldwide
–, data not determined; *, value calculated by using data from the indicated references; Time, sample collection years.
HI, hemagglutination inhibition.
Interestingly, further surveillance of IDV in North America revealed that multiple non-bovine species were susceptible to IDV. A serological survey that was performed to determine if small ruminants (sheep and goats) and poultry (chicken and turkey) were potential hosts for IDV showed that 5.2 % ( n =557) of sheep serum samples and 8.8 % ( n =91) of goat serum samples were positive for IDV antibodies, while all tested poultry serum samples, collected from multiple midwestern states of the USA, were negative for IDV antibodies ( Table 1 ) [ 18 ]. In contrast to the poultry data reported in this study, the most recent molecular diagnosis study revealed the presence of IDV genomes in poultry farms in Southeast Asia [ 29 ]. Partial sequencing of the M segment of these samples showed that the detected IDV in poultry diverged from bovine IDV strains circulating in North America. Lacking full-genome sequence information, especially the HEF sequence, prevented this study from coming to a conclusion about what genetic lineage poultry IDV belongs to. Further investigation of the susceptibility of poultry to IDV and the isolation and characterization of IDV in poultry is required to elucidate IDV infection in this species. Equines were also added to the growing host range of IDV based on serological surveillance of equine populations throughout the USA. This study showed that 15.7 % ( n =364) of equine serum samples were positive for antibodies against IDV ( Table 1 ) [ 19 ]. In America, feral swine are considered to be important vectors for IAV transmission between domestic and wild animals [ 30, 31 ]. Investigation of the seroprevalence of IDV in feral swine showed that 57 of 256 (19.1 %) samples were IDV-seropositive ( Table 1 ), which implied that feral swine might play a role in the ecology of IDV [ 32 ].
IDV in Europe
The presence of IDV in Europe was first reported in France in 2015, with the detection of 6 (4.5 %) IDV-positive samples from 134 tested cattle samples by RT-PCR during 2011–2014 ( Table 1 ) [ 33 ]. Following this initial report, IDV was subsequently found to be widespread throughout other European countries, including Italy, Luxembourg, Ireland and the UK ( Table 1 ) [ 34–37 ]. The prevalence of IDV in Europe was similar to that in North America, in which the virus and/or its specific antibodies were detected in multiple animal species, including cattle, swine, wild boar, sheep and goats, while the highest prevalence of IDV was observed in cattle ( Table 1 ) [ 36, 38–41 ]. Serosurveys in Italy, Luxembourg and Ireland revealed extremely high IDV-seropositive rates in cattle (92.4, 80.2 and 94.6 %, respectively) ( Table 1 ) [ 36, 39, 41 ]. In France, IDV was identified from cattle during 2011–2014, and a recent country-level study showed a high seroprevalence of 47.2 % ( n =3362) in cattle between 2014 and 2018, indicating that once IDV was introduced, it may have spread efficiently in cattle throughout the country ( Table 1 ) [ 33, 40 ]. Compared to the prevalence of IDV in cattle, the seropositivity rate in pigs is lower ( Table 1 ), implying that the virus is not widespread in pigs. To better understand the epidemiology and importance of IDV in the Italian swine population, serological tests were performed on 3698 swine serum samples collected in 2009 and 2015 from the same region. The results showed that in 502 sera collected in 2009, only 3 samples were positive for IDV, while 364 (11.7 %) positive samples were detected from a total of 3106 swine sera collected in 2015, suggesting that the prevalence of IDV in pigs has been increasing steadily in recent years in Italy [ 42 ].
IDV in Asia
IDV was first reported in Asia in 2014 in Shandong, PR China, with 3 IDV-positive cases (by RT-PCR) out of 453 cattle samples collected from apparently healthy cattle ( Table 1 ) [ 43 ]. A subsequent surveillance study in PR China showed a high prevalence (>30 %) of IDV in pigs and goats ( Table 1 ) [ 44 ], which was different from the relatively low prevalence found in previous studies [ 4, 18, 34 ]. Notably, this study showed that the IDV genome was present in serum samples of severely diseased cattle and goats, implying that the virus might enter transiently into the animals’ circulatory system and spread to other organs [ 44 ]. Another interesting observation from this study was that IDV was detected in goat rectal swabs, which suggested that IDV might replicate within the intestinal tract, similarly to IAV and IBV [ 44, 45 ]. The IDV genome was also found in buffalo from this study [ 44 ].
The first identification of IDV in Japan was reported from a cattle herd in the Ibaraki Prefecture in 2016 [ 46 ]. In this study, IDV showed a highly contagious property,with the virus spreading throughout the herd in a short time once the IDV infection occurred [ 46 ]. Further serological surveys indicated that IDV had been in nationwide circulation in the cattle population of Japan for at least 5 years, with an overall positivity rate of 30.5 % ( n =1267) ( Table 1 ) [ 47 ]. The positivity rates for IDV infection tended to increase with cattle age when analysing the seropositive cattle with age records at the time of collection [ 47 ]. IDV-specific antibodies were detected in samples collected as early as 2010, suggesting that IDV exposures were present in Japan from at least 2010 [ 47 ]. More recently, infection of cattle with IDV was also reported for the first time in Turkey [ 48 ].
IDV in Africa
IDV has been identified in several continents, including North America, Europe and Asia. Recently, a study reported the prevalence of IDV for the first time in Africa, by analysing total 2083 serum samples collected from cattle, swine, small ruminants and dromedary camels in Morocco, Togo, Côte d’Ivoire, Benin and Kenya during 1991–2015 [ 21 ]. According to the haemagglutination (HI) assay, this serological survey showed that IDV antibodies were detected in cattle from Morocco (2012–2015), Togo and Benin (as of 2014), and in small ruminants from Togo (2013) ( Table 1 ), suggesting that IDV had been circulating in North and West Africa since at least 2012 [ 21 ]. Significantly, the authors also showed that dromedary camels exhibited an extremely high seropositivity (99.0 %) for IDV in Kenya ( Table 1 ), indicating that camels may be a new host for IDV [ 21 ]. This work was further confirmed by another serological survey in Ethiopia, where a high seroprevalence of IDV was also observed in dromedary camels [ 49 ]. In sum, IDV infection has been demonstrated in several animal species in Africa through serological evidence, but the virus has not yet been isolated on this continent [ 21, 49, 50 ]. Future studies, such as virus isolation and antigenic characterization, are probably needed to fully illustrate the role of African animals in replication and transmission of IDV.
Genetic and antigenic evolution of IDV
Since 2011, the full genomes of more than 50 bovine and 5 swine IDV strains have been sequenced in 6 countries (the USA, PR China, France, Italy, Ireland and Japan). Phylogenetic and antigenicity analyses revealed at least four co-circulating lineages: D/OK, D/660, D/Yama2016 and D/Yama2019 ( Fig. 2 ), with ~4 % diversity for the HEF gene. Currently, in America and Europe, both D/OK and D/660 lineages are observed. These two lineages currently co-circulate in US and European cattle populations. We speculate that frequent reassortments between these two lineages in bovines may lead to the generation of new antigenic variants, which can break through the pre-existing herd immunity and pose a further threat to agricultural animal health. In PR China, only strains belonging to the D/OK lineage were reported. As demonstrated in Fig. 2 , in the D/OK lineage, strains from PR China were separated from strains from the USA and Italy, suggesting that there are sub-lineages in different countries. The swine and bovine strains within the D/OK lineage from the USA and Italy were clustered together, demonstrating that the D/OK lineage is actively transmitting worldwide between swine and bovine. Members of the D/OK lineage isolated in dairy cows, pigs and goats from Guangdong, PR China showed very low genetic diversity (genetic distance <0.005), suggesting very recent species jumping events in this location ( Fig. 2 ), but the directions of transmission are unclear. In the D/660 lineage, the strains from France and the USA diverged from each other earlier than strains within each country, suggesting that this lineage also evolved into sub-lineages in different countries. Recently, novel IDV strains that emerged in Japan have been proposed as a third lineage, D/Yama2016, and D/Yama2019, respectively ( Fig. 2 ). Interestingly, these two lineages are only present in Japan, and diverge substantially from D/OK and D/660 lineages circulating in other countries. Moreover, a novel reassortant virus was detected at a European dairy farm, with the PB2, PB1, NP, P42 and HEF segments from the D/660 genetic lineage and the P3 and NS segments from the D/OK genetic lineage [ 51 ]. These findings highlight the need to continue monitoring the prevalence of IDV to better understand its epidemiology and evolution. Our published phylogenetic analysis based on these limited data showed that IDV diverged from the other three influenza genera approximately 300 to 1200 years ago. Recently, IDV antibodies were detected in archived US cattle sera dating back to 2003–2004 [ 25 ]. This indicates that IDV has already existed for a long time. Nevertheless, little is known about the origin, rate and mode of evolution (genetic reassortment and recombination), population dynamics and migration trajectory of IDV, which warrant further investigation.

Phylogenetic tree of IDV HEF segment. Maximum-likelihood analysis in combination with 1000 bootstrap replicates was used to derive an evolutionary tree based on the nucleotide sequences of the IDV HEF segment. More than 50 % of the bootstrap values are shown next to the branches. The scale bars indicate the number of substitutions per site. Red, IDV in North America; purple, IDV in Asia; green, IDV in Europe.
Novel biology of IDV
Idv has a broad receptor binding property.
Viral attachment to receptors on the host cell surface is the initial step of the virus life cycle. Sialic acid (SA) has been found to serve as a receptor for many important human and animal pathogens, including influenza, parainfluenza, corona, noro, rota, mumps and DNA tumour viruses [ 52 ]. SA is a member of the large family of molecules derived from neuraminic acid, which is a monosaccharide containing a nine-carbon backbone, ubiquitously expressed in higher vertebrates [ 53, 54 ]. N -acetylneuraminic acid (Neu5Ac) and N -glycolylneuraminic acid (Neu5Gc) are the most common SAs found on mammalian cell surfaces, and are both ligands for influenza viruses. SAs are generally attached to glycan chains of glycoproteins or glycolipids via different glycosidic linkages. The most frequent linkage types are α2,3 linkage and α2,6 linkage to a galactose residue. IAV and IBV only recognize α2,3- or α2,6-linked sialic acid moieties as receptors ( Fig. 1 ) [ 52 ]. However, ICV utilizes N -acetyl-9- O -acetylneuraminic acid (Neu5,9Ac 2 ) ( Fig. 1b ), which has an additional O -acetyl group at the C9 position as the receptor, which is independent of α2,3 or α2,6 linkage to the adjacent galactosyl residue [ 13 ]. Structural and functional studies of the IDV HEF indicate that IDV can use both 9- O -acetylated Neu5Ac (Neu5,9Ac 2 ) and Neu5Gc (Neu5Gc9Ac), regardless of the α2,3 or α2,6 linkage, as its receptor for entry into the cell ( Fig. 1 ) [ 32, 55 ].
The functional form of the spike of influenza viruses is a homotrimer ( Fig. 3 ). The overall structures of IAV and IBV HAs are similar, despite sharing a low level of sequence homology (<30 %) [ 11 ]. During the virus life cycle, HA is primarily synthesized as a precursor (HA0) that is subsequently cleaved into a globular HA1 subunit and a stalk-like HA2 subunit [ 56, 57 ]. The major domains of the HA protein include the R domain, containing the receptor-binding pocket (RBP), the E domain, which has a vestigial esterase region, and the F domain harbouring a fusion peptide that mediates fusion between the viral and host membranes ( Fig. 3 ) [ 56–58 ]. The HEFs of ICV and IDV share ~53 % amino acid homology [ 4 ] and possess an almost identical structure and mode of ligand binding [ 13, 59 ]. Similar to HA, HEF is synthesized as a precursor (HEF0) that consists of two post-translationally cleavable life cycle: the globular head subunit HEF1 and the membrane-near stalk subunit HEF2 ( Fig. 3 ) [ 13, 59 ]. HEF also can be divided into three domains: the receptor-binding domain (R), the esterase domain (E) and the fusion domain (F) ( Fig. 3 ) [ 13, 59 ]. HEF contains the esterase activity in the E domain, while it is degenerated in HA. One interesting difference between ICV HEF and IDV HEF is that the IDV HEF protein has an open channel in the RBP, while in the structurally identical site of ICV, there is a salt bridge formed by the positively charged residue lysine (K235) and the negatively charged residue aspartate (D269) [ 59 ]. The equivalent positions of IDV HEF are threonine (T239) and alanine (A273), which cannot form the salt bridge interaction [ 59 ]. The open and flexible receptor-binding cavity of HEF may allow IDV to recognize more diverse 9-O-acetyl SAs, which further broaden its cell tropism and host range.

Schematic illustration of the HA/HEF. Linear order of the sequence fragments in HA/HEF with each individual functional domain indicated in a different monochromatic colour scheme: fusion domain (F1, F2 and F3, red); esterase domain (E1, E1’ and E2 in the HEF, blue; only E1’ in the HA, grey); receptor domain (R, green); fusion peptide (FP, black); and transmembrane region (TM, grey). The functional form of the HA/HEF is a homotrimer.
IDV has evolved a novel strategy to produce the M1 protein
Influenza viruses have evolved diverse strategies to produce viral proteins to maximize the genome coding potential. Splicing has been demonstrated in the NS and/or M segments of influenza viruses. All types of influenza viruses possess a similar mechanism to generate the NS1 and NS2 proteins ( Fig. 4 ) [ 16, 60 ]. Generally, the NS1 protein is encoded by a colinear mRNA transcript from the first initiation codon of the NS segment, while the NS2 protein is encoded by a spliced mRNA transcript from the NS segment, which shares the first portion of the NS1 transcript and then switches to the second portion within the 3′ end of the NS segment ( Fig. 4 ). However, each type of influenza viruses uses a unique strategy to produce M1 and/or M2 proteins ( Fig. 4 ). In IAV, M1 protein is made from an unspliced colinear mRNA but M2 protein is synthesized by using a spliced mRNA template [ 61 ], which is similar to the production of NS1 and NS2 proteins ( Fig. 4 ). In contrast, the M segment of IBV is not spliced. IBV M1 protein is encoded by a colinear transcript, and M2 protein is generated via a translational stop–start mechanism ( Fig. 4 ) [ 62 ]. Interestingly, ICV M1 mRNA needs to be spliced to create a termination codon, while its M2 protein is derived from the proteolytic cleavage of a P42 propeptide encoded by an unspliced colinear mRNA ( Fig. 4 ) [ 51, 63 ]. IDV uses a similar proteolytic cleavage strategy to that of ICV to generate M2 protein from the P42 protein [ 64 ], while in contrast to the ICV M segment that generates the M1 protein via splicing that only introduces a termination codon, the splicing of the IDV M segment produces an additional 4-amino-acid peptide into the preceding exon [ 16 ]. The function of these four amino acids is still unclear. Recently, a study reported that IAV M segment RNA splicing was an important host range determinant [ 65 ]. It would be interesting to speculate that different mechanisms for the production of M1 and M2 proteins among four types of influenza viruses may determine viral host range and tissue tropism, which should be tested experimentally in future studies.

Strategies used by influenza viruses to produce the NS1, NS2, M1 and M2 proteins. The NS1 protein of four types of influenza viruses is encoded by an unspliced mRNA transcript from the first initiation codon of the NS segment, while the NS2 protein is encoded by a spliced mRNA transcript from the NS segment by using the splicing mechanism. In IAV the M1 and M2 proteins are produced in a similar way to to the NS1 and NS2 proteins. In IBV, M1 protein is encoded by a colinear transcript, whereas the M2 protein is generated via a translational stop–start mechanism. In ICV and IDV, the unspliced and spliced mRNAs from the M segment encode the P42 and M1, respectively. The cleavage of the P42 by a signal peptidase produces the M1’ and M2 proteins. ICV M1 mRNA needs to be spliced to create a termination codon, while the splicing of the IDV M segment produces an additional 4-amino-acid peptide added into the preceding exon of the M1. The boxes represent different coding regions and the shapes filled with grey strips represent introns in the mRNAs. The filled cycles at the 5′ end of the mRNAs represent the 5′ cap, and the A(n) at the 3′ end represents the poly(A) tail. Other lines at both ends of the mRNAs represent noncoding regions. Modified from [ 95 ].
IDV has exceptional thermal and acid stability
IDV has been found to be the most stable of the four types of influenza viruses. Only IDV is still infectious after a 15 min exposure to 53 ℃ in neutral solution and, surprisingly, it retains infectivity even after exposure to 53 °C for 2 h [ 66 ]. Furthermore, IDV survives after being treated at pH 3.0 for 30 min, while all other types of influenza viruses are completely inactivated [ 66 ]. Recombinant IAV whose HA and NA are replaced with HEF of IDV acquires the ability to resist high-temperature and low-pH treatments, which is similar to the HEF donor virus (IDV) [ 66 ]. These observations indicate that the HEF is the primary determinant of the exceptional thermal and acid stability of IDV. Since the acid stability of HA has been found to impact on the host range, infectivity and transmissibility of IAV [ 67 ], further studies on the mechanism by which HEF confers environmental stability to IDV may offer new insights into the interspecies adaptation and transmission of the novel IDV. In addition, this unique property of IDV may partially explain why the IDV genome, but not that of IAV, IBV or ICV, could be detected in people or patients through the molecular surveillance of respiratory viruses with bioaerosol sampling in the airport or in a hospital emergency room [ 68 ].
Noncoding regions and genome packaging of IDV
Noncoding regions (NCRs) at both the 5′ and 3′ end of each segment are critical to viral genome RNA replication and subsequent genome packaging during viral egress. There is one nucleotide difference observed in the conserved 3′ noncoding ends of the vRNA segments between IDV and its closely related ICV. Specifically, the nucleotide 5 from the 3′ end is adenine (A) for IDV, while it is cytosine (C) for ICV [ 4 ]. A recent study showed that this single nucleotide difference at position 5 of the 3′ NCR within the first 11 nucleotides between IDV and ICV negatively influences the cross-recognition of the heterotypic promoter by the viral RNP complex [ 69 ]. This functional incompatibility in vRNA replication between IDV and ICV may contribute to the failure of viable reassortment between IDV and ICV. It should be noted that all segments of IDV have uracil (U) at their 3′ terminal end. 3′ terminal nucleotide U is conserved in all influenza viruses, which made it complementary to conserved 5′ terminal nucleotide A to form a high-order RNA structure that promotes influenza genome replication. Nevertheless, the NCRs across the seven segments between IDV and ICV are relatively variable in both sequence and length, especially after the first 11 to 12 nucleotides. These sequence variations, together the polymorphism at position 5 within the 3′ noncoding sequences, may have a negative impact on the efficient cross-recognition of a heterotypic promoter by the viral RNP complex, resulting in the inhibition of vRNA replication and limiting functional reassortment between IDV and ICV. Finally, each vRNA segment exists in the form of a vRNP. It is known that IAV and IBV package eight vRNPs arranged in a ‘1+7’ pattern into their virions [ 70, 71 ]. Interestingly, ICV and IDV also package eight vRNPs arranged in the ‘1+7’ pattern in spite of the fact that these two viruses only have seven genome segments [ 72 ]. The nature of the eighth vRNP observed in the virions of ICV and IDV remains unclear, which may warrant further investigation.
It is worth mentioning that two independent groups have recently developed a plasmid-based IDV reverse-genetics system. One involves a 7-plasmid system [ 69 ], while the other relies on an 11-plasmid method [ 73 ]. Viruses rescued from both systems appear to be very similar to the wild-type virus in aspects of replication, receptor binding, antigenic property and infectious titres. Despite the fact that these two RGSs were developed using the same IDV virus (D/swine/Oklahoma/1314/2011), the described reverse-genetics platform should be of great value in facilitating basic research to understand the infection biology, cross-species transmission mechanism and pathogenicity of the novel IDV.
Pathogenicity of IDV
Pathogenicity of idv in swine and cattle.
IDV is distributed worldwide and can infect multiple animal species ( Table 1 ). Two surveillance studies reported that IDV RNA was detected in pig lung samples, implying IDV infection in the lower respiratory tract [ 42, 44 ]. However, domestic pigs experimentally infected with IDV exhibited no clinical signs of illness ( Table 2 ) [ 4 ]. In addition, this study revealed that IDV infection was limited to the upper respiratory tract of pigs ( Table 2 ) [ 4 ]. Interestingly, an infection experiment conducted on feral swine showed that IDV was able to replicate in both the upper and lower respiratory tract, including nasal turbinate, soft palate, trachea and lung tissues. Nevertheless, no clinical signs of the respiratory disease were observed ( Table 2 ) [ 32 ]. Intriguingly, IDV viraemia was observed in the infected feral swine [ 32 ]. A recent animal study indicated that IDV infection caused lung lesions in pigs possessing IAV-specific antibodies [ 74 ]. These conflicting reports in terms of viral tropism in swine may be caused by animal genetics, experimental conditions and the virus strains used in studies. Despite the discordance in tropism, all these animal studies indicated that IDV was transmissible among pigs by direct contact [ 4, 32, 74 ]. Further studies are needed to clarify the pathogenesis and transmission of IDV in swine because influenza disease can be readily transmitted from swine to humans and other animal species.
Replication, pathogenicity and transmission of IDV in experimentally infected animals
‘ na ’ indicating that data not available.
Cattle are the primary reservoir for IDV. Several surveillance studies on bovines showed that IDV RNA was detected more frequently in those with respiratory diseases, including pneumonia, than in healthy controls [ 24, 27, 44, 75 ]. Several metagenomic studies performed on cattle with BRD found a positive correlation between BRD and IDV infection [ 27, 28, 75 ]. However, appreciable levels of IDV RNA were also detected in apparently healthy cattle [ 24, 36, 41 ]. To further determine the pathogenesis and transmission of IDV, several experimental studies were conducted with IDV-negative cattle ( Table 2 ). These animal studies found that IDV caused mild respiratory diseases in experimentally infected cattle [ 76–79 ]. Two of these studies demonstrated that IDV replicated in both the upper and lower respiratory tract [ 77, 78 ]. Inflammations characterized by neutrophils were observed in the nasal turbinate [ 76 ], trachea [ 76, 77 ], or bronchial lumens [ 78 ]. Importantly, these studies also confirmed that IDV transmitted between cattle through direct contact or aerosol [ 77, 78 ]. Overall, cattle, as the natural reservoir of the virus, play an important role in IDV replication and dissemination, which emphasizes the need for continued monitoring and risk assessment for this emerging virus in bovines.
As stated above, IDV is highly associated with BRD, which is caused by complex interactions between the host, infectious agents and environmental factors [ 80 ]. Generally, cattle with stress and under adverse environmental conditions are more susceptible to a primary viral infection and the compromised host immunity caused by the viral infection probably facilitates a secondary bacterial invasion of the respiratory tract, which often result in severe illness and even death in cattle [ 79, 80 ]. Viruses, including bovine viral diarrhoea virus, infectious bovine rhinotracheitis virus, bovine respiratory syncytial virus, parainfluenza type-3 virus and the newly identified IDV, and bacteria such as Mannheimia haemolytica (MHA), Pasteurella multocida , Histophilus somni and Mycoplasma bovis , are considered to be important pathogens for BRD [ 27, 28, 75, 79 ]. To determine the potential role of IDV in BRD, an animal study was designed for cattle involving coinfection with IDV and MHA. The results showed that primary IDV infection in cattle did not enhance the disease caused by the secondary MHA infection [ 79 ]. Interestingly, this result was similar to that of an independent mice study, which found that IDV infection in mice did not increase the susceptibility to secondary Staphylococcus aureus infection, but was protective against clinical signs of the secondary bacterial infection [ 81 ]. More studies are needed to define synergistic or antagonistic actions behind the opportunistic infection with IDV and determine the underlying mechanisms of IDV pathogenicity in IDV-associated coinfection.
Pathogenicity of IDV in other animals
The pathogenicity and transmissibility of IDV were also determined by experimental infection of ferrets, guinea pigs and mice ( Table 2 ). These laboratory animals are widely used in influenza A and B research. Ferrets have been used as a surrogate for influenza virus infection of humans. No clinical signs of disease were reported in these animals infected with IDVs [ 4, 81–83 ]. In ferrets, IDV replicated in the nasal turbinate but not in the lower respiratory tract [ 4 ]. The virus could be transmitted to naïve ferrets by direct contact [ 4 ]. In contrast to ferrets, IDV in guinea pigs was detected in both the upper and lower respiratory tract, and could also be transmitted to the sentinels by direct contact [ 83 ]. IDV replication in mice was observed mainly in the upper respiratory tract but also to a lesser extent in the lower respiratory tract [ 82 ]. Importantly, the virus was detected with low titres in the mouse intestine [ 82 ], which was in agreement with a recent study that found IDV in rectal swabs of goats [ 44 ]. This enteric tropism of IDV seems to be supported by a study showing the exceptional acid stability of IDV when compared to other types of influenza. It may be interesting to test whether IDV can use a faecal–oral transmission route to replicate and transmit among animals.
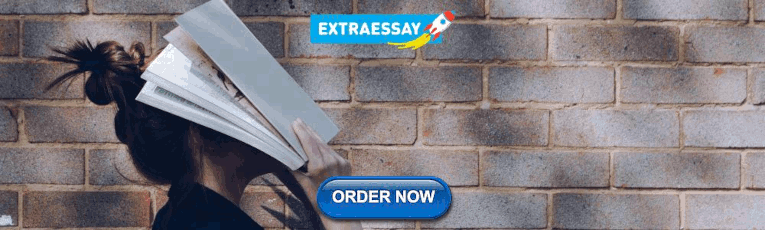
Zoonotic potential of IDV
There are no indications so far that IDV can cause and transmit disease in humans. IDV is an emerging influenza virus that has a broad host range, which is similar to IAV. As mentioned above, IDV can infect and/or be transmitted to multiple domestic animals, including cattle, pigs, sheep, goats, camels, horses and poultry, and also to wild species such as feral pigs. In addition, IDV is able to replicate and transmit by direct contact in ferrets and guinea pigs, both surrogate models for human influenza [ 4, 83 ]. A recent study confirmed that IDV was able to efficiently replicate in the well-differentiated human airway epithelial cells (hAECs) that served as an in vitro respiratory epithelium model for humans [ 84 ]. This model has been well applied to assess the zoonotic potential of emerging respiratory viruses [ 85, 86 ]. Generally, successful zoonotic infections occur when the pathogens acquire the ability to cross the species barrier and replicate efficiently in a novel host [ 87 ]. Taken together, these findings suggest that multiple-species-infecting IDV has great zoonotic potential to infect humans.
Several lines of genetic evidence further supported the above hypothesis. First, an aerosol surveillance of respiratory virus genomes at the Raleigh-Durham International Airport observed that among 4 of the 24 samples positive for known respiratory pathogens, 3 tested positive for adenovirus and 1 was positive for IDV [ 68 ]. Second, the IDV genome was detected in another molecular surveillance of respiratory pathogens with bioaerosol sampling in a hospital emergency room setting in North Carolina, USA [ 88 ]. Third, a earlier study involving the same approach also showed that the IDV RNA genome was detected in nasal wash samples of a swine farm worker in Malaysia [ 89 ]. These studies demonstrated that humans who tested positive for IDV had been exposed to the virus. In contrast to these aerosol sample-based surveillance studies, in a clinical investigation involving the traditional RT-PCR approach no IDV genomes were detected in more than 3000 patient respiratory samples in Scotland, UK [ 90 ].
In parallel, several serological studies were conducted in North America and Europe, and these works demonstrated that IDV-specific antibodies were present in humans. An earlier serosurvey in the USA showed that 1.3 % ( n =316) of the general human population had antibodies against IDV [ 4 ], Notably, in a more recent study, a very high seroprevalence of IDV was observed among workers with cattle exposure history (34 out of 35 people had IDV antibodies), as reported in a serological study in Florida, USA [ 20 ]. In addition, a more comprehensive seroprevalence study in Italy revealed that IDV antibodies were present and increased over time in the Italian population from 2005 to 2017 [ 91 ]. The results showed that low levels of antibodies against IDV were found in the years 2005–2007 (5.1 %–9.8 %), but these increased sharply in the years 2008–2010 (37.9–43.4 %) and 2013–2014 (41.0–46.0 %), followed by a decline in the subsequent years 2011–2012 and 2015–2017 [ 91 ]. Interestingly, this longitudinal study revealed a temporal correlation between IDV prevalence peak in humans and epidemics in domestic pigs in Italy. The prevalence of antibodies against IDV in humans implies that the virus may infect humans and pose a potential threat to human health.
It should be noted that the primary serology assay utilized in the above antibody surveillance studies is the traditional haemagglutination inhibition (HI) assay, which is used routinely to titrate the antibody response to influenza infection. The antibodies the HI assay measure are those that block a functional interaction between the virus and the sialic acid receptor and as a result neutralize virus infection. HI assays involving virus-specific hyperimmune sera generated in rabbits, guinea pigs and sheep revealed no detectable cross-reactivity between IDV and ICV, as reported in several studies [ 19, 20, 91 ]. Nevertheless, some level of cross-reactivity between IDV and ICV was observed in the HI assay when ferret sera were used. Since ferret is a great surrogate model for human influenza, and ICV is a ubiquitous human pathogen, a concern has been raised about the significant contribution of non-specific cross-reactivity from ICV to the IDV antibody prevalence data obtained in humans when the HI is only assay used in serological investigations. As such, development of a virus-specific assay without the cross-reactivity is urgently needed to accurately evaluate IDV antibody prevalence in humans and assess its risk to human health.
Influenza D virus (IDV) was first identified in 2011 from a clinically ill pig in the USA. In nearly a decade since its appearance, the virus has been detected in multiple animal species worldwide and represents an important concern to animal health. IDV utilizes cattle as a primary amplification host and a potential reservoir. The viral infection can cause mild respiratory disease in cattle and has been indicated as a causative agent of bovine respiratory disease (BRD) complex, which is the most common and costly disease affecting the global cattle industry. Importantly, outbreaks of IDV in swine and bovine are increasing, and increasing genetic and serological evidence is showing that IDV has the potential to adapt to humans. IDV has also evolved some novel biological characteristics. One of the most interesting traits is that IDV is the most stable of the four types of influenza viruses. The underlying mechanisms that contribute to the unique biology and cross-species transmission of IDV are still not fully understood. The recent development of robust reverse-genetics systems for IDV [ 69, 73 ] will facilitate future studies aiming to advance our understanding of the biology of IDV at the molecular level and illuminate the viral determinants driving cross-species transmission.
Funding information
This study was supported by NIH R01AI141889, SDSU-AES 3AH-673, USDA/NIFA 2016-67016-24949, NSF/EPSCoR ( http://www.nsf.gov/od/iia/programs/epscor/index.jsp ) award IIA-1335423, the SD-CBRC supported by the State of South Dakota’s Governor’s Office of Economic Development, University of Kentucky AES, and William Robert Mills endowment fund to University of Kentucky.
Acknowledgements
We thank all the members of the Li and Wang laboratories for their input into this work. We also thank SDSU’s functional genome core facility for providing equipment and resources for our experiments.
Conflicts of interest
The authors declare that there are no conflicts of interest.
Abbreviations: BRD, bovine respiratory disease; HEF, hemagglutinin-esterase-fusion; HI, hemagglutination inhibition; IDV, influenza D virus; Neu5,9Ac 2 , N -acetyl-9- O -acetylneuraminic acid; Neu5Gc9Ac, 9-O-acetylated N-glycolylneuraminic acid; SA, sialic acid.
Thank you for visiting nature.com. You are using a browser version with limited support for CSS. To obtain the best experience, we recommend you use a more up to date browser (or turn off compatibility mode in Internet Explorer). In the meantime, to ensure continued support, we are displaying the site without styles and JavaScript.
- View all journals
- Explore content
- About the journal
- Publish with us
- Sign up for alerts
- Published: 28 June 2018
- Florian Krammer 1 ,
- Gavin J. D. Smith 2 , 3 ,
- Ron A. M. Fouchier 4 ,
- Malik Peiris 5 , 6 ,
- Katherine Kedzierska 7 ,
- Peter C. Doherty 7 , 8 ,
- Peter Palese 1 , 9 ,
- Megan L. Shaw 1 ,
- John Treanor 10 ,
- Robert G. Webster 11 &
- Adolfo García-Sastre 1 , 9 , 12
Nature Reviews Disease Primers volume 4 , Article number: 3 ( 2018 ) Cite this article
118k Accesses
796 Citations
164 Altmetric
Metrics details
- Influenza virus
- Respiratory tract diseases
- Viral pathogenesis
Influenza is an infectious respiratory disease that, in humans, is caused by influenza A and influenza B viruses. Typically characterized by annual seasonal epidemics, sporadic pandemic outbreaks involve influenza A virus strains of zoonotic origin. The WHO estimates that annual epidemics of influenza result in ~1 billion infections, 3–5 million cases of severe illness and 300,000–500,000 deaths. The severity of pandemic influenza depends on multiple factors, including the virulence of the pandemic virus strain and the level of pre-existing immunity. The most severe influenza pandemic, in 1918, resulted in >40 million deaths worldwide. Influenza vaccines are formulated every year to match the circulating strains, as they evolve antigenically owing to antigenic drift. Nevertheless, vaccine efficacy is not optimal and is dramatically low in the case of an antigenic mismatch between the vaccine and the circulating virus strain. Antiviral agents that target the influenza virus enzyme neuraminidase have been developed for prophylaxis and therapy. However, the use of these antivirals is still limited. Emerging approaches to combat influenza include the development of universal influenza virus vaccines that provide protection against antigenically distant influenza viruses, but these vaccines need to be tested in clinical trials to ascertain their effectiveness.
Introduction
Influenza is an infectious respiratory disease; in humans, it is caused by influenza A (genus influenzavirus A ) and influenza B (genus influenzavirus B ) viruses ( influenzavirus C and influenzavirus D genera are also known). Symptoms associated with influenza virus infection vary from a mild respiratory disease confined to the upper respiratory tract and characterized by fever, sore throat, runny nose, cough, headache, muscle pain and fatigue to severe and in some cases lethal pneumonia owing to influenza virus or to secondary bacterial infection of the lower respiratory tract. Influenza virus infection can also lead to a wide range of non-respiratory complications in some cases — affecting the heart, central nervous system and other organ systems 1 , 2 . Although characterized by annual seasonal epidemics, sporadic and unpredictable global pandemic outbreaks also occur that involve influenza A virus strains of zoonotic origin. Pandemic influenza occurs every 10–50 years and is characterized by the introduction of a new influenza A virus strain that is antigenically very different from previously circulating strains; the lack of pre-existing immunity in humans is often associated with the severity of the infection and an increase in mortality.
All influenza viruses are enveloped negative-sense single-strand RNA viruses with a segmented genome. Influenza A and influenza B viruses contain eight RNA segments, which encode RNA polymerase subunits, viral glycoproteins (namely, haemagglutinin (HA), with its distinct globular ‘head’ and ‘stalk’ structures, which facilitate viral entry, and neuraminidase (NA), which facilitates viral release), viral nucleoprotein (NP), matrix protein (M1) and membrane protein (M2), the nonstructural protein NS1 and nuclear export protein (NEP) (Fig. 1 ). The HA and NA viral proteins are the most antigenically variable, and in the case of influenza A virus, they are classified into antigenically diverse subtypes. These two viral glycoproteins are located at the surface of the virus particle and are the main targets for protective antibodies induced by influenza virus infection and vaccination. Each influenza virus isolate is named according to its type or genus, host and place of isolation, isolate number and year of isolation (Box 1 ). Influenza C and influenza D viruses have only seven RNA segments and do not seem to cause substantial disease in humans. However, influenza C virus infections can cause influenza-like illness and hospitalizations in some instances, especially in children 3 .
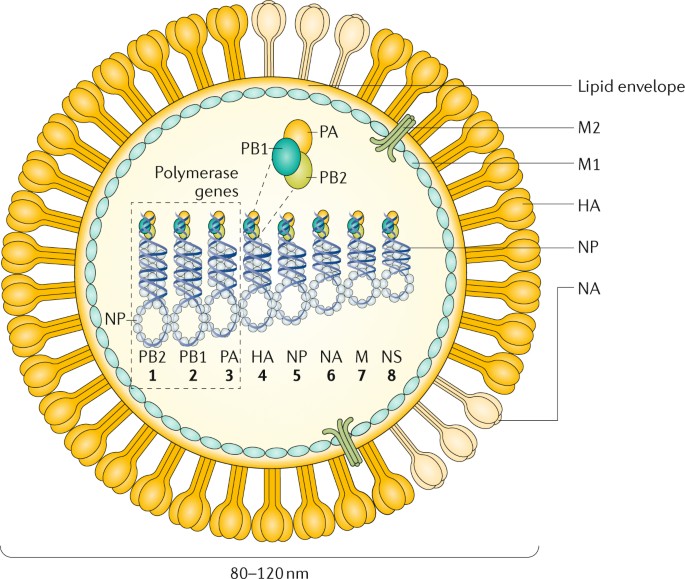
The figure represents an influenza A virus particle or virion. Both influenza A and influenza B viruses are enveloped negative-sense RNA viruses with genomes comprising eight single-stranded RNA segments located inside the virus particle. Although antigenically different, the viral proteins encoded by the viral genome of influenza A and influenza B viruses have similar functions: the three largest RNA segments encode the three subunits of the viral RNA-dependent RNA polymerases (PB1, PB2 and PA) that are responsible for RNA synthesis and replication in infected cells; two RNA segments encode the viral glycoproteins haemagglutinin (HA, which has a ‘stalk’ domain and a ‘head’ domain), which mediates binding to sialic acid-containing receptors and viral entry, and neuraminidase (NA), which is responsible for releasing viruses bound to non-functional receptors and helping viral spread. The RNA genome is bound by the viral nucleoprotein (NP), which is encoded by RNA segment 5. RNA segments 6 and 8 encode more than one protein, namely, the matrix protein (M1) and membrane protein (M2) — BM2 in the case of influenza B — and the nonstructural protein NS1 (not shown) and nuclear export protein (NEP). The M1 protein is thought to provide a scaffold that helps the structure of the virion and that, together with NEP, regulates the trafficking of the viral RNA segments in the cell; the M2 protein is a proton ion channel that is required for viral entry and exit and that, together with the HA and NA glycoproteins, is located on the surface of the virus anchored in a lipid membrane derived from the infected cell. Finally, the NS1 protein is a virulence factor that inhibits host antiviral responses in infected cells. The influenza viruses can also express additional accessory viral proteins in infected cells, such as PB1–F2 and PA-x (influenza A), that participate in preventing host innate antiviral responses together with the NS1 protein or NB (influenza B), the function of which is unknown. NS1, NEP, PB1–F2 and PA-x are not present in the virus particle or are present in only very small amounts. NB is a unique influenza B virus surface protein anchored in the lipid membrane of the virus particles.
The segmented nature of the influenza viral genome enables reassortment, that is, interchange, of genomic RNA segments when two viruses of the same type (that is, two influenza A viruses or two influenza B viruses) infect the same cell. A unique characteristic of influenza A viruses is that they circulate not only in humans but also in domestic animals, pigs, horses and poultry and in wild migratory birds (>100 species of ducks, geese, swans, gulls, waders and wild aquatic birds are considered natural reservoirs 4 , 5 ). A total of 16 antigenically different HA and 9 antigenically different NA serotypes or subtypes (18 HA and 11 NA, if including bat influenza A-like viruses, which are phylogenetically close to influenza A virus but unable to reassort with influenza A viruses 6 , 7 ) have been identified among the different avian strains of influenza A virus 8 . These animal reservoirs provide a source of antigenically diverse HA and NA genes that can be exchanged between viral strains by reassortment after co-infection of the same host, increasing virus diversity and in some instances leading to the generation of human pandemic influenza virus strains with HA and/or NA derived from animal strains. The HA subtypes can be grouped into two groups on the basis of the phylogeny of the HA molecule. Within each group, the domain encoding the HA stalk is antigenically similar. By contrast, influenza B and influenza C viruses are not divided into different subtypes and are restricted to humans, with no known animal reservoirs, although limited spillover to seals and pigs has occurred, respectively 9 , 10 . However, influenza B viruses have recently diverged into two antigenically different lineages (B/Victoria/2/1987-like and B/Yamagata/16/1988-like) that are currently co-circulating in humans, which prompted the inclusion of these two lineages, in addition to the influenza A H1N1 and H3N2 viruses, in many of the seasonal human influenza vaccines. Influenza C viruses are usually associated with very mild or asymptomatic infections in humans. In addition, influenza D virus is distantly related to influenza C virus, and it has been isolated from pigs and cows 11 , 12 .
In 2009, the first influenza virus pandemic of this century was caused by a novel H1N1 influenza A virus reassortant that was previously circulating in pigs 13 , 14 . Vaccines were not available on time to contain the first waves of infection. Current influenza antiviral adamantane drugs that target the viral M2 ion channel and inhibitors of NA enzymes have limitations, as viral resistance against M2 inhibitors is prevalent in the currently circulating human influenza A H1N1 and H3N2 strains; viruses resistant to the NA inhibitor oseltamivir have been prevalent among influenza A H1N1 strains since just before the 2009 pandemic 15 . Intriguingly, the adamantane-resistant, oseltamivir-sensitive 2009 pandemic influenza A H1N1 viruses replaced the previously circulating oseltamivir-resistant, adamantane-sensitive seasonal influenza A H1N1 virus that circulated globally in 2008–2009. Although detection of the currently circulating influenza A H1N1 viruses harbouring the NA-H275Y mutation, which imparts oseltamivir resistance, has been noted, emergence of resistance to NA inhibitors is not a major public health problem today 16 . Current seasonal influenza vaccines have only suboptimal effectiveness across all age groups, particularly in elderly individuals (a high-risk group for severe influenza virus infection). Thus, there is a major need to better understand the biology of influenza virus infections to develop new and more-effective antiviral agents and vaccines.
In this Primer, we discuss the biological features of influenza viruses and their effects on human and animal health, as well as the mitigation strategies to reduce the burden of influenza. We focus more on influenza A viruses because of their unique pandemic potential compared with other influenza viruses.
Box 1 Influenza virus nomenclature
Each influenza virus isolate receives a unique name according to a set of rules. First, the name denotes the type of influenza virus (A, B, C or D), followed by the host species from which the virus was isolated (if not specified, the isolate is considered human), the geographical location at which the virus was isolated, the isolate number and the year of isolation. In the case of influenza A viruses, the haemagglutinin (HA) and neuraminidase (NA) subtype is also usually indicated after the viral isolate name. For example, influenza A/Turkey/Ontario/6118/1968 (H8N4) virus is an influenza A virus isolated from a turkey in Ontario in 1968, isolate number 6118; the virus isolate has an HA from the HA antigenic subtype 8 and an NA from the NA antigenic subtype 4.
Epidemiology
Influenza in humans.
The major burden of disease in humans is caused by seasonal epidemics of influenza A and influenza B viruses, with most of the infections occurring in children, although most of the severe cases involve very young or elderly individuals. Seasonal influenza A H1N1 and H3N2 currently circulate in humans, but influenza A H2N2 viruses were the only human circulating influenza A viruses from 1957 to 1968. Before 1918, conclusive evidence of the circulating subtypes is not available. In addition to seasonal epidemics, the introduction of influenza A viruses from either avian or swine populations has led to four pandemics since 1918, with those viruses subsequently becoming seasonal epidemic strains in subsequent years (Fig. 2 ). During pandemics, influenza viruses spread very quickly from the point of origin to the rest of the world in several waves during the year (Fig. 2 ) owing to the lack of pre-existing immunity, which could also contribute to increased virulence. Studies on the transmission of seasonal influenza A virus in humans have proposed that populations in southeast Asia, eastern Asia and/or the tropics act as permanent sources for seeding seasonal epidemics 17 , 18 , whereas other studies indicate that multiple geographical regions might act as seed populations for virus migration 19 . However, a lack of sufficient sequence data from areas such as Africa, India and South America currently prevents a complete understanding, as viral sequences, which are not routinely obtained during diagnosis, are required to ascertain the relationships between viruses spreading in different areas of the world. Seasonal influenza virus outbreaks typically occur in the winter months, when low humidity and low temperatures favour transmission; two ‘influenza seasons’ occur per year: one in the Northern Hemisphere and one in the Southern Hemisphere. However, unlike temperate regions, seasonal influenza patterns are very diverse in tropical countries. Climate factors, including minimum temperature, hours of sunshine and maximum rainfall, seem to be the strongest predictors of influenza seasonality 20 . Seasonal influenza B viruses co-circulate in humans with influenza A viruses and follow the same patterns of transmission.
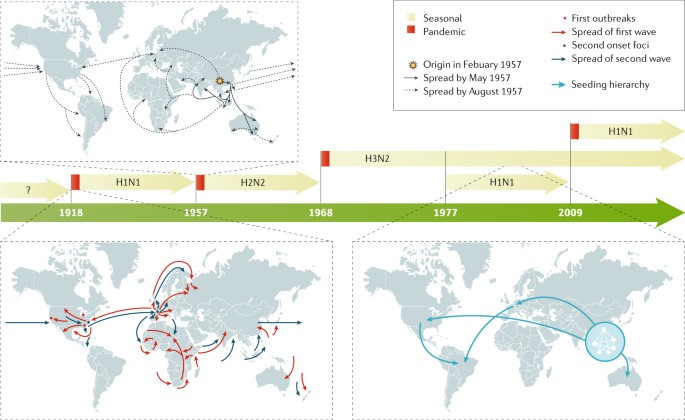
In the past 100 years, four pandemics of human influenza have occurred, with the 1918 pandemic caused by an influenza A H1N1 virus being the most devastating, as it was associated with >40 million deaths 236 . Influenza A H2N2, H3N2 and H1N1 viruses caused the 1957, 1968 and 2009 pandemics, respectively. In 1977, influenza A H1N1 restarted circulation in humans without causing a pandemic, as the strain was similar to that which preceded the 1957 influenza A H2N2 pandemic. By contrast, the 2009 pandemic influenza A H1N1 virus was antigenically very different to the previous seasonal influenza A H1N1 viruses and replaced them as the circulating influenza A H1N1 strain. Examples of the spreading of human influenza A viruses in the world are shown for pandemic 1918 and 1957 viruses and for seasonal H3N2 viruses 18 . For pandemic virus outbreaks, the arrows indicate the first and second waves of transmission. For seasonal influenza A H3N2 spread, the arrows indicate the seeding hierarchy of seasonal influenza A (H3N2) viruses over a 5-year period, starting from a network of major cities in east and southeast Asia; the hierarchy within the city network is unknown. Seasonal influenza B viruses (not shown) are co-circulating in humans with influenza A viruses.
In the United States and from 2010 to 2017, the Centers for Disease Control and Prevention (CDC) has estimated that influenza virus infection has resulted in between 9.2 million and 35.6 million illnesses and between 140,000 and 710,000 hospitalizations 21 . In a typical year, 3–5 million cases of severe illness are caused by seasonal influenza virus infection in the world. Children seem to be the main human transmitters of influenza viruses, as illustrated by studies showing that vaccination of children reduces the incidence of severe influenza virus infections in elderly individuals 22 . Incidence of influenza virus infections increases during pandemic years owing to the lack of pre-existing immunity against the new virus, but severity varies depending on the pandemic virus itself, with the 1918 influenza A H1N1 pandemic being the most severe of the past 100 years.
Zoonotic human infections with influenza A virus strains of swine and avian origins are rare, with specific strains, such as the avian H5N1 and H7N9 and the so-called swine H3N2 variant viruses, being the most prevalent but confined to a limited number of human cases in specific geographical areas, with only limited human-to-human transmission. Prior age-related immunological imprinting in specific age groups acquired by exposure to viruses of the same or related subtype is associated with reduced incidence of disease with novel subtype influenza viruses 23 .
Influenza A and influenza B viruses cause epidemic seasonal infections, resulting in ~500,000 deaths annually worldwide 24 , with the most recently calculated estimates being 291,243–645,832 deaths per year during the 1999–2015 period 25 . Seasonal influenza virus-associated deaths in the United States range from 5,000 to 52,000 people per year, depending on the year 26 . Several comorbidities are known to increase the risk of lethal influenza virus infection. In addition, pregnancy and age are known risk factors, with very young (<1 year of age) and elderly (>65 years of age) individuals being the most vulnerable populations. Although mortality is low in children >2 years of age and young adults, a substantial number of deaths owing to influenza virus infection have been recorded in young individuals with no known predisposition factors. Approximately 100 children die each year from influenza in the United States; this number has been stable since 2010 (ref. 27 ). Severe disease and/or mortality in patients with influenza virus infection are in general due to either virus-induced pneumonia or secondary bacterial superinfection. Primary viral pneumonia is characterized by high levels of viral replication in the lower respiratory tract accompanied by strong pro-inflammatory responses, also referred to as cytokine storm.
Risk factors
Young children who have not had multiple previous exposures to influenza viruses are fairly susceptible to infection and typically have higher fever and more-severe symptoms and shed larger number of virus particles for longer periods of time. Older adults, either because of immunosenescence, concurrent chronic illnesses or both, are also at risk of more-severe illness, hospitalization and infectious complications 28 . In addition to the extremes of age, other groups at risk of severe disease, hospitalization or death include those with chronic pulmonary or cardiac conditions, diabetes mellitus or immunocompromising conditions 29 , 30 . Possibly because of difficulty handling respiratory secretions, individuals with neuromuscular disorders are also recognized as being at increased risk 31 . Pregnancy has long been recognized as a risk factor for severe influenza disease, possibly because of the increased cardiopulmonary demands of pregnancy or the effects of pregnancy on the immune system; the risk increases by trimester and persists into the post-partum period 32 . During the 2009 pandemic with influenza A H1N1, morbid obesity with body mass index >35–40 kg per m 2 was also recognized as an important risk factor 33 . Obesity is associated with excessive influenza virus replication, increased severity of secondary bacterial infections and reduced vaccination efficacy 34 . Finally, the role of host genetic factors in disease remains poorly studied, but polymorphisms and nonsense mutations in several host genes (such as IFITM3 and IRF7 , which encode genes involved in the interferon pathway) have been shown to predispose to severe disease 35 , 36 , 37 .
Influenza in animals
Infection of waterfowl with low pathogenic avian influenza A (LPAI) viruses, called as such owing to their lack of lethality in poultry, produces little or no clinical symptoms 38 . However, upon transmission to poultry, LPAI can cause substantial disease symptoms; in addition, viruses with H5 and H7 subtypes can become highly pathogenic (that is, lethal) in poultry. Outbreaks of highly pathogenic avian influenza A (HPAI) throughout Eurasia and northern Africa are the major influenza disease burden in animals, with >400 million birds killed and economic losses totalling US$20 billion in the first 10 years of the 21st century 39 . Outbreaks of HPAI in poultry have also caused considerable problems in the United States, with the HPAI H5N x (where x = 1–9) viruses, derived by reassortment of the Asian HPAI H5N1 viruses, being a recent example 40 . Recently, LPAI H7N9 viruses circulating in China have become HPAI through the acquisition of a multibasic cleavage site in their HAs and have also caused some self-limiting infections in humans 41 . Influenza A viruses can also cause mild to severe respiratory infection in a variety of mammals, including swine, horses and dogs; additionally, an outbreak of an influenza A virus of avian origin was detected in cats in New York, as first reported in December 2016. From its first report until February 2017, the influenza A H7N2 feline influenza A virus infected ~500 shelter cats and a veterinarian who treated one of the infected cats 42 .
Mechanisms/pathophysiology
Human influenza viruses are transmitted through the respiratory route, whereas avian influenza viruses in wild birds are transmitted through the faecal–faecal, faecal–oral or faecal–respiratory routes. Depending on the route of transmission, the virus targets epithelial cells of the respiratory or intestinal tract for infection and productive replication. In addition, some avian influenza A viruses, especially those of the H7 subtype, have been associated with human infections of the eye and conjunctivitis (inflammation of the conjunctiva) 43 . Severity of infection in humans is associated with replication of the virus in the lower respiratory tract, which is accompanied by severe inflammation owing to immune cell infiltration.
Transmission in animals
The main reservoir of diverse strains and subtypes of influenza A virus is wild birds, especially migratory ducks and geese. Indeed, domestic ducks raised on open ponds are the logical intermediaries between the reservoirs of influenza viruses in wild aquatic birds and other domestic poultry (Fig. 3 ). Transmission between birds can occur directly from contaminated water. Although most of the 16 HA and 9 NA influenza A subtypes cause asymptomatic infections in avian species, H5N x and H7N x subtypes can evolve into HPAI strains through the acquisition of a novel cleavage site in HA (which enables HA maturation by ubiquitous host proteases and spread of the virus outside the respiratory and intestinal epithelia to multiple organs, including the brain) and can cause lethal infections in chicken, turkey and some breeds of domestic duck 44 , 45 . Transmission of influenza viruses to multiple domestic poultry species occurs through so-called backyard farming, whereby species are raised together and in live poultry markets; subsequent transmission to commercial avian farms can occur through the lack of biosecurity (that is, procedures or measures designed to isolate and protect animals and humans from contact with infectious viruses) and because of viral spread through live markets 46 (Box 2 ).
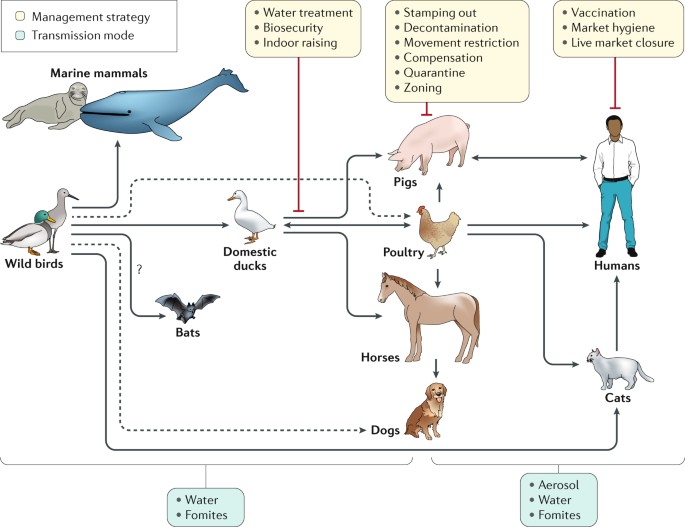
Influenza A viruses have been found in multiple species all seemingly derived from viral ancestors in wild birds, with the possible exception of bat influenza-like virus, which is of still uncertain origin. Influenza viruses from wild birds can spill over through water or fomites to marine mammals and to domestic free-range ducks. Transmissions to other avian species (for example, poultry) from domestic ducks or directly from wild birds can also occur from contaminated water. Transmission from ducks to other species occurs through ‘backyard’ farming, whereby the animals are raised together, and in live poultry and/or animal markets. Transmission from backyard to commercial farms can occur via lack of biosecurity and via spread through live markets 46 . Humans can be infected with poultry and swine influenza viruses through aerosols, fomites or contaminated water. However, in most instances these infections do not result in subsequent human-to-human transmission. Human-to-human transmission of seasonal or pandemic human viruses can be mediated by respiratory droplets, aerosols or self-inoculation after touching of fomites. Additional virus adaptations would be required for sustainable human-to-human transmission of animal influenza viruses. Other domestic animals known to be susceptible to influenza virus infections are dogs and cats. Dashed lines represent transmission that bypasses a domestic duck intermediate.
Influenza in pigs is a respiratory disease akin to influenza in humans, with high fever and pneumonia caused by influenza A H1N1, H3N2 and H1N2 subtypes 47 . Some strains of influenza A virus have been known to be transmitted by aerosol spread from humans to pigs and vice versa, including the pandemic 2009 influenza A H1N1 strain 48 and the influenza A H3N2 variant that transmits from pigs to children 49 . In horses, infection causes respiratory disease and is spread by aerosol; two lineages of influenza A H3N8 are primarily responsible. In 2004, the equine influenza A H3N8 strain spread to dogs 42 , 50 . In 2006 in Asia, an avian H3N2 influenza A virus was also detected as being transmitted to dogs 51 .
Box 2 Avian (poultry) influenza
The influenza viruses causing disease outbreaks in domestic poultry are the highly pathogenic avian influenza A (HPAI) H5N x and H7N x (where x = 1–9) and the low pathogenicity avian influenza A (LPAI) H6N1, H7N9 and H9N2 strains 228 . The major global problem for farmers of gallinaceous poultry (for example, chicken, quail and turkey) is the emergence of H5 and H7 HPAI viruses from LPAI precursors that must be reported to the World Organisation for Animal Health (OIE) and can result in trade embargoes. In poultry, the HPAI strains are transmitted both by aerosol and faecal contamination and cause systemic haemorrhagic disease and death 229 . Between 2003 and 2017, the HPAI H5N1 strain infected 860 humans and caused 545 deaths 230 . The LPAI H7N9 strain that emerged in China in 2013 causes asymptomatic infections of quail and chicken 231 , but from 2013 to 2017, a total of 1,564 laboratory-confirmed human infections with this virus were reported in China 232 . To complicate matters, some LPAI H7N9 viruses have recently become HPAI 233 . But perhaps the most insidious influenza virus in domestic poultry on the basis of its prevalence and ability to reassort is H9N2, which emerged in Asia in the early 1990s; this virus causes asymptomatic infection in poultry, was the donor of six internal RNA segments to both HPAI H5N1 and LPAI H7N9 and was occasionally transferred to pigs and humans 234 , 235 .
Cell entry and viral replication
At the cellular level, influenza virus replication (Fig. 4 ) mainly takes place in epithelial cells of the respiratory tract in humans and other mammals and in epithelial cells of the intestinal tract in birds. The cellular cycle of the virus starts with binding to the target cell. This binding is mediated by the viral HA (which binds to sialic acids present in the oligosaccharides of the glycoproteins at the cellular surface) and is also responsible for the haemagglutination caused by virus particles when incubated with red blood cells. HAs from human influenza viruses bind preferentially to sialic acids linked by an α2,6 linkage to the rest of the oligosaccharide, whereas those from most of the avian influenza viruses favour binding to α2,3-linked sialic acids, as these bonds are the most abundant sialic linkages in the epithelial cells of the human upper respiratory tract and of the avian intestinal tract, respectively 52 , 53 . After binding, the virus is internalized in an endosome, and the endosome is trafficked and acidified, which triggers a conformational change in the viral HA that induces the fusion of the viral envelope with that of the endosome. As the endosomal pH varies between host species, the pH stability of HA is one of the determinants of viral tropism.
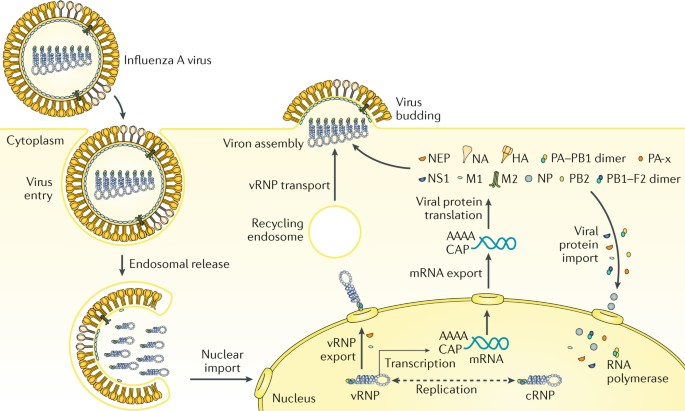
Influenza virus enters the cell by endosomal uptake and release, and its negative-sense genetic material in the form of viral ribonucleoproteins (vRNPs) is imported to the nucleus for transcription of mRNA and replication through a positive-sense complementary ribonucleoprotein (cRNP) intermediate. Viral mRNA is translated into viral proteins in the cytoplasm, and these are assembled into new virions together with the newly synthesized vRNPs. PB1–F2 is shown here as a dimer, but can also be multimeric. HA, haemagglutinin; M1, matrix protein; M2, membrane protein; NA, neuraminidase; NEP, nuclear export protein; NP, nucleoprotein; NS1, nonstructural protein; PB1, PB2 and PA, viral RNA polymerases.
Fusion results in the release of virus contents, namely, its genetic material in the form of eight viral ribonucleoproteins (vRNPs), into the cytoplasm. vRNPs are subsequently imported into the nucleus of the infected cells, where transcription and replication of the viral RNA takes place through the enzymatic activities of the viral polymerase complex attached to the vRNPs. Viral RNA replication occurs through a positive-sense intermediate, known as the complementary ribonucleoprotein (cRNP) complex. Viral RNA transcription results in positive-strand mRNAs that are capped and polyadenylated and exported into the cytoplasm for translation into viral proteins. Newly synthesized viral polymerases (PB1, PB2 and PA) and viral NP are imported to the nucleus to further increase the rate of viral RNA synthesis, whereas virus membrane proteins HA, NA and M2 traffic to and get inserted into the plasma membrane. Newly synthesized HA needs to be cleaved into HA1 and HA2 polypeptides by cellular proteases to be functional. The cleavage site of HA is responsible for the tissue tropism of the virus, with all influenza viruses having a cleavage site recognized by extracellular proteases present in respiratory and intestinal epithelial cells, except for the HPAI viruses, which contain a multibasic cleavage site in HA that is recognized by ubiquitous proteases. Viral nonstructural proteins NS1, PB1–F2 (which can be dimeric or multimeric) and PA-x regulate cellular processes to disarm host antiviral responses. Viral M1 and NEP localize to the nucleus at late stages of viral infection, bind to vRNPs and mediate their export to the cytoplasm, where, through interactions with the recycling endosome, they migrate to the plasma membrane and are bundled into the eight vRNPs. Budding of new virions takes place, resulting in the incorporation of the vRNPs into new virus particles with a membrane derived from the host plasma membrane and containing the viral transmembrane proteins. NA activity prevents non-productive binding of HA of new virions to receptors bearing sialic acid present in the viral glycoproteins and in the membrane of the infected cells, facilitating viral spread. Viral replication results in cell death with pathological implications. In addition, viral products induce a pro-inflammatory response that is responsible for the recruitment of innate and adaptive immune cells, which clear and eliminate the virus, but that in excess induces immunopathology and pneumonia.
Antigenic drift
Influenza viruses are capable of evading the antibody-mediated immunity induced during previous infections or vaccinations by gradually accumulating mutations in HA and NA 5 (Fig. 5 ). This process, known as antigenic drift, necessitates frequent updates of influenza vaccines to ensure sufficient antigenic relatedness between the vaccine and emerging virus variants. Because HA is currently the main component of the inactivated influenza vaccines, human and animal influenza virus surveillance programmes are in place to monitor antigenic changes of the virus, primarily using the haemagglutination inhibition assay (a surrogate assay to measure the inhibition of the interaction between HA and host cell receptors) and viral RNA sequence analyses 54 , 55 . Increased attention to antigenic drift of NA, measured using neuraminidase inhibition assays, could further increase the antigenic match between vaccines and circulating viruses 56 , 57 , 58 . In addition, antigenic drift of T cell epitopes has been observed but not as frequently as antibody-mediated drift 59 . Computational methods, so-called antigenic cartography, enable quantitative analyses and visualizations of haemagglutination inhibition assay data and neuraminidase inhibition assay data 60 .
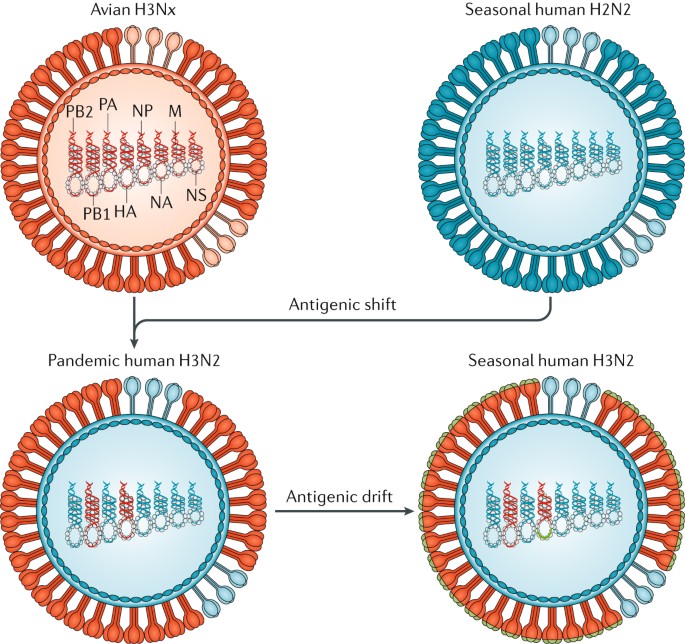
In 1968, co-infections between an avian influenza A H3N x (where x = 1–9) virus and the seasonal human influenza A H2N2 viruses resulted in the exchange of viral segments (reassortment) and the selection of the pandemic human influenza A H3N2 virus, with the RNA polymerase PB1 and haemagglutinin (HA) RNA segments derived from the avian virus and the rest of the segments derived from the human virus. The lack of pre-existing immunity to the antigenically novel H3 HA in humans facilitated human transmission. Similar reassortment processes have taken place during other influenza A virus pandemics. Once H3N2 became established in humans, the virus began to drift, as is the case with all other human seasonal influenza viruses. During drift, small antigenic changes in the HA protein generated by mutation are selected to increase immune evasion, although not as dramatically as during shift. M, RNA encoding M1, matrix protein, and M2, membrane protein; NA, RNA encoding neuraminidase; NP, RNA encoding nucleoprotein; NS1, RNA encoding nonstructural protein; PB2 and PA, RNA encoding RNA polymerases.
These methods to quantify antigenic drift of influenza virus strains, along with site-directed mutagenesis studies, have revealed that substantial antigenic drift can be caused by one or very few amino acid substitutions adjacent to the functional sites in the head (but not the stalk) of the HA protein 61 , 62 , 63 . The antigenic evolution of influenza viruses was shown to be more rapid in human influenza viruses than in swine and equine viruses, which is presumably related to the size, mixing and age-structure (that is, humans have a longer lifespan than swine and horses) of the host populations 60 , 63 , 64 . In wild birds, in which numerous avian influenza A virus HA and NA subtypes are circulating, within-subtype antigenic variation seems to be limited. However, upon introduction in poultry, antigenic variation within avian virus subtypes — perhaps related to a selective antibody pressure imposed by vaccine use — has been noted 62 .
Antigenic shift
In contrast to antigenic drift, antigenic shift refers to drastic changes in the antigenicity of the HA of circulating influenza A viruses; antigenic shift is associated with influenza A pandemics. The HA — and sometimes the NA — molecules of pandemic viruses are derived from antigenically diverse animal strains of influenza virus, which can be acquired by human influenza strains through reassortment (Fig. 5 ). Pandemic outbreaks are usually associated with the extinction of the previous circulating strains. However, in 1977, influenza A H1N1 viruses, not seen in humans since the 1957 influenza A H2N2 pandemic, started to co-circulate with influenza A H3N2 viruses 65 . The 2009 influenza A H1N1 pandemic was caused by an influenza A H1N1 virus that was antigenically very different from the seasonal influenza A H1N1 virus circulating at the time and resulted in the extinction of the previous influenza A H1N1 human lineage, but it did not result in the extinction of the influenza A H3N2 viruses. Since 2009, influenza A H3N2 and influenza A H1N1 viruses derived from the 2009 pandemic virus and two lineages of influenza B virus are co-circulating in humans.
The limited number of pandemic events that have happened makes it very difficult to predict the next pandemic. Human influenza A virus infections with antigenically diverse avian H5N1, avian H7N9, swine H3N2 and other animal influenza viruses are constantly detected in geographical regions where these strains are prevalent owing to the contact of infected poultry or swine with humans. However, no cases of sustained human-to-human transmission have been associated with these viruses, indicating that further adaptations need to take place for these viruses to become transmissible in humans. How feasible it is for these viruses to become adapted to human transmission and retain virulence is unclear. Only a few changes are needed for influenza A H5N1 viruses to become transmissible in ferrets, a host that is often used as an animal model of human influenza virus infection 66 , 67 , 68 .
Adaptations to new hosts
Aquatic waterfowl (for example, orders Anseriformes and Charadriiformes) host influenza A viruses of all the HA (H1–H16) and NA (N1–N9) subtypes and are believed to be the natural reservoirs of influenza viruses 69 . Some viruses, presumably from waterfowl, have adapted to other birds (Galliformes, such as chicken, quail and turkeys) and mammals (such as humans, swine, equine, dogs, mink and marine mammals) 70 . Such inter-species transmission and adaptation often involve viral genetic adaptations to the new host and can be attributed to viral RNA polymerases, which are error prone and have higher mutations rates than cellular DNA polymerases. The ensuing intra-host viral genetic diversity (sometimes referred to as viral quasi-species) results in a ‘swarm’ of viral mutants that facilitate adaptation to new selection pressures. Multiple genetic variants are transmitted between animals, and low-fitness variants can be maintained during transmission, presumably helped by the fitter variants within a host 71 . Maintenance of such genetic diversity in the virus population within one host would be potentially advantageous in adaptation following inter-species transmission events. In addition, the segmented RNA genome of the influenza virus enables viral genetic reassortment, providing an additional mechanism for genetic adaptation.
Adaptation to transmission in new hosts is usually multifactorial and could involve amino acid substitutions in the viral HA to optimize receptor binding 72 , to optimize HA and NA balance between their receptor binding and their receptor destroying activities, respectively 73 , and to enable HA to become activated at different pH values 74 . Additionally, changes in the viral RNA polymerase protein subunits can affect its activity in different hosts and affect the temperature sensitivity of the virus 75 , 76 (the temperature of the human upper respiratory tract is ~33 °C, which is lower than that of birds and swine); changes in NP can affect susceptibility to the host antiviral protein interferon-induced GTP-binding protein Mx1 (ref. 77 ), and changes in M1 and M2 proteins could alter viral morphology and facilitate respiratory transmission in new hosts 78 . Such functional changes that affect transmission could also be acquired through viral genetic reassortment, as occurred with the emergence of the human pandemics of 1957, 1968 and 2009 (ref. 79 ).
Genetic adaptations can occur at the interface between wild aquatic birds and domestic ducks or geese, between aquatic and terrestrial poultry (as occurred with the emergence of avian influenza A viruses H5N1, H7N9 and H10N8) or within swine (as occurred with the emergence of the North American triple reassortant influenza A viruses and of the 2009 pandemic influenza A H1N1 virus). Of the influenza A H3N8 and H7N7 viruses endemic in equine for many decades, the H7N7 viruses seem to have become extinct, whereas the H3N8 viruses have persisted and have been transmitted to dogs in the United States 50 , increasing the host range of H3N8 viruses. In another example, avian influenza A H3N2 viruses have adapted to dogs in Asia 51 . Recently, species of bat from Guatemala and Peru have been found to carry influenza A-like viruses with new subtypes of HA and NA (namely, H17, H18, N10 and N11), and further influenza virus diversity can be detected in bats 6 , 80 . Although bat influenza A-like H17N10 and H18N11 viruses are unable to reassort with conventional influenza A viruses, they can replicate in mammalian species other than bats, raising the possibility that they could become adapted to other mammals 81 .
Innate immune responses
Host innate immunity represents a crucial barrier that viruses need to overcome to replicate and propagate in new hosts, and influenza viruses dedicate several viral proteins to overcome these responses. For example, the NS1 protein of the virus is an RNA-binding protein that prevents the activation of cytoplasmic viral RNA sensors, such as retinoic acid-inducible gene I protein (RIG-I; also known as DDX58) 82 . RIG-I recognizes the influenza viral RNAs on the basis of the presence of a terminal 5′-triphosphate moiety and an adjacent double-stranded RNA structure formed by the 5′ and 3′ ends of the viral RNAs that is required for viral RNA replication and transcription 83 . NS1 binds to the host factors E3 ubiquitin-protein ligase TRIM21 and E3 ubiquitin-protein ligase RNF135 (also known as Riplet), which are required for RIG-I activation after viral RNA recognition, preventing a signalling cascade that otherwise leads to induction of interferon and interferon-inducible antiviral genes with antiviral activity, such as MX1 , EIF2AK2 (more commonly known as PKR ), OAS1 , IFITM family members and IFIT family members, among others 84 . Moreover, influenza A virus NS1 can also prevent host mRNA synthesis, processing and trafficking events, inhibiting host responses to viral infection 85 , 86 , 87 . Interferon signalling events mediated by the Janus kinase (JAK)–signal transducer and activator of transcription (STAT) pathway as well as the antiviral functions of several interferon-stimulated genes might also be targeted by NS1 to prevent the host antiviral response 88 , 89 , 90 .
In addition to NS1, several other influenza virus proteins can dampen the interferon-mediated antiviral response. PB1–F2, a viral nonstructural protein generated from an alternative open reading frame present in the PB1 RNA, suppresses the activation of mitochondrial antiviral-signalling protein (MAVS), an adaptor molecule located downstream of RIG-I and required for interferon induction 91 . PB2, a component of the viral polymerase, also seems to target MAVS activation 92 . PA-x, a recently discovered viral protein resulting from ribosomal frameshift of the viral RNA polymerase PA mRNA, suppresses host gene expression by virtue of its RNA endonuclease activity 93 . The multiple ways in which influenza virus counteracts the interferon-mediated antiviral response illustrate the importance of this host pathway.
Both type I interferons (namely, interferon-α (IFNα) and IFNβ) and type III interferons (namely, IFNλ) can inhibit viral replication in epithelial cells 94 . Tissue-resident macrophages and dendritic cells can also be infected by the virus, but viral production seems to be limited in these innate immune cells 95 . Nevertheless, exposure of these cells to viral infection results in their activation and the secretion of pro-inflammatory cytokines, leading to the recruitment of additional innate cells, such as natural killer cells and pro-inflammatory monocytes, to the lungs, where they can control viral infection by killing infected cells. Although these host responses to infection are crucial for final viral clearance and induction of adaptive immune responses, an exacerbated response can lead to immunopathology and severe disease. For instance, high levels of neutrophil extracellular traps (chromatin-based structures released from neutrophils) can lead to lung damage and severe influenza virus infection 96 . Therapies aimed to reduce this so-called cytokine storm might be beneficial for patients with severe influenza 97 .
Adaptive immune responses
T cell immunity.
Although mouse models are not considered optimal for analysing the pathogenesis of viral pneumonia, much of the research into how influenza virus-specific T cells work has used such systems. However, as the technology for monitoring T cell-mediated immunity in humans has improved, these basic insights have been validated 98 , 99 , 100 , 101 , 102 . In influenza virus infection, CD4 + T cell help is required for class-switching of antibodies and for optimal CD8 + T cell memory responses 103 , 104 . CD4 + T cell help is also required for peptide-based (and other) vaccines to promote optimal humoral and cellular immunity 105 , 106 . It is less clear whether CD4 + T cells are important effectors of direct influenza virus clearance that work via IFNγ production 99 , 104 , as occurs in infections with large DNA viruses such as herpesviruses.
With respect to CD8 + T cells, cytotoxic T lymphocyte (CTL) effectors are required for optimal influenza virus clearance 107 , 108 . In their absence, antibody-mediated mechanisms might also work, though less rapidly. Prominent antigenic components are the NP, matrix protein M1 and the viral polymerases 109 . Of particular interest is the fact that infected cells display viral peptides 8–12 amino acids in length that bind major histocompatibility complex (MHC; encoded by the HLA genes) class I glycoproteins to form the antigenic complex (epitope) recognized by T cell receptors on influenza virus-specific CTLs. These peptides are generally derived from components inside the virus that are less subject to antibody-selected antigenic drift and, therefore, are more conserved across distinct influenza virus strains and subtypes. Strong evidence supports the idea that established CTL memory directed towards conserved viral peptides presented by widely prevalent human MHC glycoproteins (for example, that are encoded by HLA-A2 ) can provide a measure of protection against a novel influenza A virus that crosses over from an avian reservoir to humans 101 (for example, the recent H7N9 cases 101 ) (Fig. 6 ). Furthermore, the increased influenza virus susceptibility of some indigenous populations (in Australia and Alaska) correlates with the relatively low frequency of such ‘protective’ HLA types within these populations 100 .
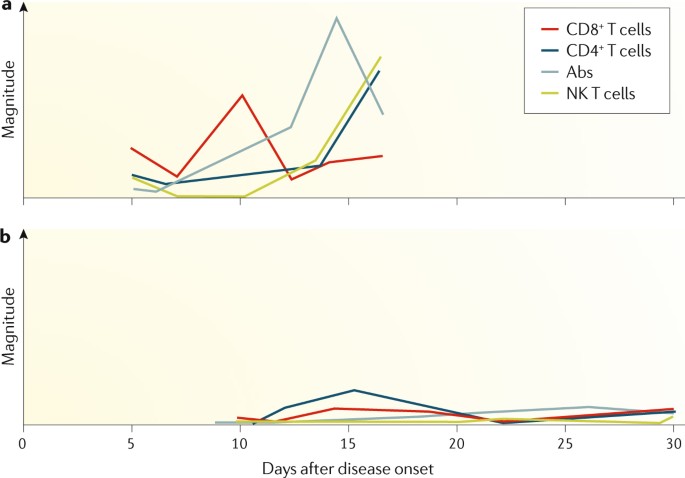
a | Patients who recovered early from severe influenza A H7N9 disease had rapid and prominent CD8 + cytotoxic T lymphocyte (CTL) recall responses. These early interferon-γ (IFNγ)-producing CTLs were most likely derived from pre-existing memory pools established after seasonal human influenza A virus infection, which then cross-reacted with H7N9. CTL responses were followed by antibody (Abs) responses 2–3 days later and by CD4 + T cells and natural killer (NK) cell responses 101 . b | By contrast, individuals who succumbed to severe influenza disease had minimal cellular or antibody immunity.
Given the unpredictability of influenza A virus pandemics and the inevitable lag phase in the production of vaccines (which are typically inactivated influenza virus vaccines), there is, of course, broad interest in possible strategies for cross-protective immunization. Live attenuated vaccines certainly have the potential to induce CD8 + T cell responses, but evidence to date suggests that they do not promote strong CTL memory 110 . Strategies aimed at maintaining large numbers of influenza virus-specific CTLs in the normal lung may both be impractical and have physiological consequences; instead, effector CTLs will likely need to be recalled from the recirculating ‘resting’ CTL memory pool. This approach can give more rapid virus clearance than is the case in a CTL-naive individual, resulting in milder disease outcomes.
Major questions are what constitutes optimal CTL memory, and how is this established. At a basic level, epigenetic profiles of the various CTL differentiation states (naive, effector and memory) are being defined to better understand the processes involved 111 . Also, as naive CTL sets are lost with age, priming influenza virus-specific CTLs early is important 112 . As for many other questions with CTLs, analysis of influenza virus infections will continue to inform the overall understanding of protective viral immunity.
B cell immunity
B cell responses are an integral part of the immune response to both influenza virus infection and influenza virus vaccines. Antibodies contribute substantially to the protection from infection, as shown by early experiments in which sera from an influenza virus-immune ferret could protect a naive ferret from influenza disease after challenge 113 . B cell responses mainly target viral HA and NA and, to a lesser degree, other viral proteins, including the NP and the matrix proteins 114 . Of note, discrete antigenic sites on the head domain of HA seem to be the preferential target of the antibody response 115 ; these sites are immunodominant over most other B cell epitopes of the virus 116 , 117 . However, the molecular and immunological reasons for antigenic immunodominance remain obscure. Antibodies that target the head domain of HA are typically neutralizing (via blockade of receptor binding) and show inhibitory activity in the haemagglutination inhibition assay. Importantly, the haemagglutination inhibition titre correlates (imperfectly) with protection and is a surrogate marker for vaccine efficacy that is globally accepted by regulatory agencies 118 .
Antibody responses to influenza virus infection in naive humans are typically robust and long lasting. For example, antibodies raised to strains encountered in childhood can usually be detected at reasonable levels in elderly individuals and might protect from virus variants with similar antigenicity encountered later in life 119 , 120 , 121 , 122 . However, antigenic drift particularly affects the HA head domain, which has high plasticity 123 , 124 . Over the course of a lifetime, individuals might be exposed to or infected by influenza viruses several times. Interestingly, the first infection in life and its immune imprinting might skew antibody responses to subsequent exposures. This phenomenon was originally described as the original antigenic sin 125 , but different aspects of it have been called antigenic seniority, antigenic imprinting and back-boosting 126 . Typically, exposure to a new virus variant (or subtype) might also induce antibodies to viruses encountered earlier in life, depending on the relatedness of the two antigens. An antibody response against a novel virus variant in adults might, therefore, be heavily influenced by the individual’s exposure history 117 , 127 , 128 , which is an issue for vaccine development.
In addition to neutralizing strain-specific antibodies, viral infection can also induce low-level humoral immune responses against conserved epitopes of viral proteins 126 . Among these epitopes are the membrane proximal stalk domain of HA 129 , 130 , the extracellular domain of the M2 protein 131 and conserved epitopes of NA 132 , 133 , 134 , 135 . The broad antibody responses against these epitopes might contribute to protection, and they often work through mechanisms that rely on crystallizable fragment (Fc)–Fc receptor (FcR) interactions (effector functions) or — in the case of anti-NA antibodies — NA inhibition 136 , 137 , 138 . However, the antibody responses induced by such conserved epitopes through infection or immunization (with current vaccines) are weak and reduce their potential contributions to protection in the general population.
As the mucosal surfaces of the respiratory tract are the main entry pathway for influenza viruses in humans, secreted antibodies play a major part in the prevention of infection. Key experiments in the guinea pig model of influenza have shown that only mucosal immune responses (including immunoglobulin A (IgA)) but not systemic immune responses can efficiently inhibit virus transmission 139 , 140 , which is important considering that influenza virus vaccines should prevent both disease in the host and transmission.
Diagnosis, prevention and screening
A diagnosis of influenza is made mostly on the basis of clinical presentation and epidemiological likelihood of infection. Initially, nonspecific symptoms predominate, including fever, chills or frank shaking, headaches, myalgia (muscle pain), malaise (discomfort) and anorexia. The onset of these symptoms is sudden, and respiratory symptoms, particularly a dry cough, sore or dry throat (possibly with hoarseness) and nasal obstruction and discharge, are usually also present. Cough is the most frequent respiratory symptom and can be accompanied by substernal discomfort or burning. Presentation in older adults and in individuals with compromised immune systems can initially be less dramatic, possibly because of a diminished cytokine response, but initial mild symptoms can progress to severe lower respiratory disease in these patients. The presentation can include fever, lassitude (lack of energy) and confusion without the characteristic respiratory complaints, which may not occur at all. Presentation in children can include febrile seizures but other prominent systemic complaints may not be present.
During seasonal influenza epidemics, diagnosis on the basis of clinical presentation has reasonable accuracy in previously healthy young and middle-aged adults, in whom the presence of fever with either cough or sore throat is associated with influenza virus infection in ~80% of patients 141 . However, as these symptoms are similar to those caused by other respiratory pathogens, which include parainfluenza virus, respiratory syncytial virus, human metapneumovirus, adenoviruses, rhinoviruses and coronaviruses, specific diagnostic tests are required to confirm infection by an influenza virus. Confirmation is important for reasons that include management of individual patients, public health responses and surveillance efforts 142 , 143 , 144 , but as patients do not always present to health-care facilities, the number of confirmed diagnoses is under-reported. The type of sample submitted for testing is usually a nasopharyngeal swab, a nasal wash or a combined throat and nasal swab; because the sensitivity correlates with viral load, samples obtained within 3 days of symptom onset are preferable.
As influenza-like illness can be caused by many viruses, the gold standard laboratory tests used for influenza are viral culture and reverse transcription PCR (RT-PCR) (Table 1 ). Virus culture is critical for detailed characterization of novel viruses, surveillance of sensitivity to antiviral drugs and monitoring of antigenic drift; it is time consuming, but the length of the test can be reduced if shell-vial co-cultures are used, which detect virus-positive cells by immunostaining before the presence of cytopathic effect 145 . By contrast, RT-PCR, which is a highly sensitive and specific molecular test, is quick, can be incorporated into multiplex assays and can be used to subtype viruses. The nucleic acid amplification tests are clearly superior to virus culture in both sensitivity and speed in the context of clinical management. For both viral culture and RT-PCR, the quality of the sample is important, as irregularities in handling can lead to degradation and false-negative results. The site sampled can also affect the sensitivity of the assay; nasopharyngeal swabs are preferred to throat swabs for detection of the virus during upper respiratory infection. As neither of these assays provides a point-of-care diagnosis, rapid antigen tests have been developed that are fast, can be run in a physician’s office and are less expensive, although low and variable sensitivity is a limitation. To overcome this limitation, a rapid molecular assay has been developed and approved by the US FDA, with results comparable to those of RT-PCR except in samples with low viral loads 146 . New point-of-care digital immunoassays also have superior sensitivity in detecting influenza A and influenza B virus infections compared with traditional rapid antigen tests 147 . Several recent point-of-care nucleic acid amplification tests with even higher sensitivities 147 are now approved in United States. Future developments might include the use of transcriptomic approaches to diagnose both the cause and the severity of respiratory illnesses, for example, the presence of secondary bacterial infections and/or inflammatory markers 148 .
After the causative agent of influenza was identified to be a virus in the 1930s, attempts were made during World War II to develop inactivated virus as a vaccine, which culminated in the licensing of the first influenza virus vaccine for the civilian population in 1945 in the United States. Unfortunately, it soon became clear that the vaccine did not protect well against new influenza virus strains. Specifically, the 1947 seasonal epidemic strain had changed sufficiently that the vaccines made with earlier circulating strains had lost their effectiveness 149 . All currently available influenza virus vaccines are injected intramuscularly, with the exception of the live attenuated influenza virus vaccines, which are administered intranasally.
Design and manufacture
Although antigenic shift and antigenic drift in influenza viruses are recognized problems, the procedure to manufacture influenza virus vaccines has remained largely unchanged for many decades. The manufacture of influenza virus vaccines involves growing the virus in embryonated chicken eggs and inactivation using formalin (or another alkylating reagent). In the 1970s, two changes were introduced. First, reassortment of up to six internal RNA segments of the high-yielding influenza A/Puerto Rico/8/1934 strain with RNA segments encoding HA and NA of the seasonal vaccine candidates improved the growth characteristics of the virus in embryonated eggs with obvious advantages for vaccine production (Fig. 7 ). Second, the whole virus is now subjected to treatment with ether or detergent to make subvirion (or split) preparations, which are much less reactogenic and are more tolerable for the patient (reviewed in ref. 150 ).
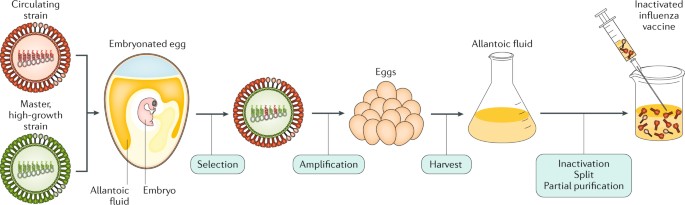
An antigenically representative circulating strain is reassorted with the high-growth influenza A/Puerto Rico/8/1934 (PR8) virus strain by co-infection in eggs, and a vaccine virus is selected with the high-growth properties of PR8 (conferred by its RNA segments encoding internal viral proteins) and the haemagglutinin (HA) and neuraminidase (NA) derived from the circulating strain. The vaccine virus is amplified in eggs, and the allantoic fluid from the infected eggs containing high titres of the virus is harvested; the virus is inactivated, treated for splitting of individual viral components and subjected to partial purification to enrich for the HA (and NA) viral components in the final injectable vaccine.
In 2003, MedImmune (Gaithersburg, Maryland, USA) introduced the first live attenuated influenza virus vaccine (LAIV) licensed in the United States. This vaccine is based on the backbones of the influenza A/Ann Arbor/6/1960 and influenza B/Ann Arbor/1/1966 strains, through the passaging of the viruses at low temperature (25 °C). Cold-adapted influenza virus vaccines based on the same principle and developed by the Institute of Experimental Medicine (IEM, Saint Petersburg, Russia) have been successfully used in Russia since 1987 (ref. 151 ). Seasonal vaccines are made by reassortment of the six internal RNA segments of the cold-adapted strain with the HA and NA RNA segments of the influenza virus strains specified by the health authorities for inclusion in the seasonal vaccines. Vaccine strain selection for both inactivated vaccines and LAIVs is based on the determination of the prevalence of recent human circulating strains by multiple laboratories engaged in a WHO-sponsored influenza surveillance programme (Box 3 ). LAIVs seem to have advantages in inducing mucosal and more broadly protective immune responses in infants and children than inactivated influenza virus preparations (reviewed in ref. 150 ). However, studies with LAIVs in the United States have shown poor efficacy against the influenza A H1N1 component 152 .
Tissue culture cell-based substrates other than embryonated eggs have the advantage of not becoming limiting in cases when vaccine production needs to increase, such as during pandemic outbreaks. Cell-grown influenza virus vaccines based on Madin–Darby canine kidney (MDCK) and Vero cells (from kidney epithelial cells of monkeys) were licensed in Europe in 2007 and 2009, respectively. However, production of the Vero-cell vaccine has been stopped, as the company that developed them ceased its vaccine production portfolio, and the FDA only approved the first MDCK-grown influenza virus vaccine in 2012 (ref. 153 ). This approval was a major accomplishment after exhaustive studies to demonstrate that the cells did not contain adventitious viruses that were due to known naturally occurring dog viruses, such as oncogenic papilloma and retroviruses.
Another breakthrough for influenza vaccines came in 2013, when the FDA licensed Flublok (Protein Sciences Corporation, Meriden, Connecticut, USA), which is a product made exclusively using recombinant DNA technologies. For the quadrivalent product, four different baculoviruses expressing the HA of two influenza A viruses and the HA of two influenza B viruses are used to infect continuous insect cell lines; the HA proteins are then extracted and purified from the infected cells — a process that completely avoids the use of influenza viruses and of embryonated eggs. Thus, there is now a faster start-up possible for vaccine manufacturing in cases of vaccine shortages or the emergence of novel pandemic strains. Also, there is no need for time-consuming adaptations of the vaccine strains to increase yields in embryonated eggs or in tissue culture, as the recombinant baculoviruses express different HAs at comparable levels in insect cells 154 .
Addition of adjuvants to inactivated influenza vaccines can improve vaccine efficacy and the breadth and duration of protection and can have dose-sparing benefits in case of years with vaccine shortages. Indeed, in November 2009, German regulatory authorities approved an MF59-adjuvanted, MDCK-grown influenza A H1N1 pandemic vaccine 155 . MF59 is a squalene-based adjuvant that increases the immunogenicity of protein-based vaccines, and MF59-adjuvanted inactivated influenza vaccines are now approved for use in people >65 years of age, in whom unadjuvanted inactivated influenza vaccines perform poorly. In 2013, the FDA approved an adjuvant influenza vaccine for the prevention of pandemic influenza A H5N1. This vaccine is for use in people >18 years of age who are at increased risk of infection with avian influenza A H5N1 viruses. The adjuvant used, AS03, similar to MF59, is a squalene-based oil-in-water emulsion that is combined with the H5N1 component before intramuscular injection. AS03-adjuvanted pandemic influenza virus vaccines have previously been used in Europe during the 2009 influenza A H1N1 pandemic, where they received close attention owing to several reported cases of vaccine-associated narcolepsy 156 , a chronic neurological disorder characterized by excessive daytime sleepiness. Contributory roles of the pandemic virus or the vaccine antigens in these adverse events could not be excluded.
To increase the poor immunogenicity of inactivated influenza vaccines in elderly individuals, an unadjuvanted high-dose vaccine has also been developed and is proven to decrease severe influenza virus infection outcomes compared with standard-dose vaccines 157 . In addition, some available influenza vaccines are now tetravalent, incorporating both influenza B virus lineages. Thus, the variety of approved influenza virus vaccines has been dramatically increased in recent decades globally. However, all these available seasonal influenza virus vaccines still necessitate annual reformulations to match antigenically circulating vaccines (Box 3 ). In addition, the short duration of immunity is another major shortcoming of the current vaccines.
Reverse genetics technologies to generate recombinant influenza viruses with defined genetic sequences from plasmid DNA were introduced in 1990 (ref. 158 ). These techniques should improve both live and inactivated influenza virus vaccine manufacturing by creating vaccine backbones with increased replication properties and stability for use in embryonated eggs or in tissue culture. Other approaches are aimed at inducing protective immune responses against the aforementioned conserved epitopes of various viral proteins. Novel and safe adjuvants based on Toll-like receptor-mediated immune stimulation and novel modes of delivery may dramatically change the vaccine field in the future. The ultimate goal of these approaches is to make an influenza virus vaccine that provides lifetime (or at least multi-year) protection, protects against all influenza antigenic variants and establishes a degree of herd immunity. Indeed, consensus is that influenza virus vaccines could and should be improved and that efforts to design a long-lasting universal influenza virus vaccine effective against different antigenic variants are worthwhile 159 , 160 .
Box 3 Seasonal influenza vaccine strain identification
Year-round surveillance for human influenza is conducted by >100 designated national influenza centres around the world. The laboratories send isolated viruses for genetic and antigenic characterization to five WHO Collaborating Centres for Reference and Research on Influenza, which are located in the United States, the United Kingdom, Australia, Japan and China. A WHO committee reviews the results of surveillance and laboratory studies twice per year and makes recommendations on the composition of the influenza vaccine on the basis of the use of antigenically matched viruses with those that are expected to be highly prevalent in the next season. Each country then makes their own decision about which viruses should be included in influenza vaccines licensed in their country.
Schedules and vaccination programmes
Vaccine recommendations vary from country to country, with some recommending vaccination in only the high-risk groups and contacts. Currently, the public health authorities in the United States and the American Academy of Pediatrics recommend yearly vaccination with seasonal influenza virus vaccine to all people >6 months of age 161 . Stronger recommendations are given to individuals in high-risk groups for severe influenza and to caregivers and contacts of those at high risk. The WHO recommends seasonal influenza vaccination to pregnant women (highest priority) and, in no particular order of priority, to children 6 months to 5 years of age, elderly individuals (>65 years old), individuals with specific chronic medical conditions and health-care workers.
In the absence of a universal vaccine that covers all possible strains and subtypes of influenza virus, many countries have implemented stockpiling vaccine programmes to store vaccines against influenza virus strains with pandemic potential for emergency first responders to alleviate the problem imposed by the rapid spread of pandemic influenza. Although preparing for potential pandemic threats is sound public health policy, it is not compelling to assume that the influenza A H5N1 viruses (or the more recently detected H7N9 virus (March 2013)) are pandemic precursor strains because they are associated with the largest numbers of human infections among animal influenza A virus strains. First, over the past 130 years, only viruses expressing an H1, H2 or H3 HA have been responsible for seasonal or pandemic strains. Avian H5N1 and H7N9 viruses have most likely circulated for at least as long without spilling over into humans and starting a new pandemic 162 . Accordingly, these ‘avian’ HA influenza viruses are unlikely to easily transmit from person to person. Second, the high case fatality rates cited by the WHO for H5N1 and H7N9 viruses in humans seem to be an artefact of the way they were calculated (that is, counting only patients who were hospitalized as having been infected by these viruses); many more people seem to have been infected by these viruses without severe outcomes 163 , 164 . Additionally, severe disease only results from exposure to large quantities of the virus, which can occur when people are in direct contact with diseased poultry. However, transmission of seasonal or pandemic influenza viruses requires only small quantities of virus (estimates vary between 2–25 virions 165 and 50–400 virions 166 ) to cause severe disease and transmission.
Efficacy and effectiveness
Despite the availability of seasonal and pandemic influenza vaccines, debate is ongoing as to the efficacy (as measured by randomized controlled trials) and effectiveness (as measured by observational studies involving vaccinated and unvaccinated individuals) of these vaccines 167 . Although different methodologies, the variability of the virulence of different seasonal strains, the fit or matching of the vaccine with the circulating strains and age differences of different cohorts can make interpretation of results between studies difficult, most studies find a positive effect of vaccination on the overall health of vaccinated individuals 168 , 169 . Nevertheless, many challenges still remain, such as the documented lack of efficacy of LAIVs in the United States in the past years 170 , the possible reduced vaccine efficacy with repeated annual immunizations 171 and the problems associated with vaccine mismatches 172 . Assays to monitor antigenicity with respect to prior viruses are performed on a regular basis, generally by government laboratories. Antigenicity of a virus is characterized by infecting naive ferrets with the cultured virus and collecting the sera 2–3 weeks later; the ability of the sera to inhibit haemagglutination compared against the current vaccine is monitored. Sera from an antigenically novel virus will show reduced haemagglutination inhibitory activity compared with previous virus strains, indicating antigenic drift and a potential vaccine mismatch.
Together with vaccines, antiviral drugs play a vital part in the prevention and treatment of influenza virus infection and disease. In a normal influenza season, antiviral drugs are primarily used for treating patients who are severely ill, particularly those who have a compromised immune system. In a pandemic setting, especially in the period before a vaccine is available, antiviral drugs are essential for both treating patients who have been infected and preventing infection in individuals who have been exposed. Two classes of drugs are currently approved for influenza, namely, the adamantanes and the NA inhibitors.
Amantadine and rimantadine are both orally administered drugs that target the M2 ion channel of influenza A viruses. However, these drugs are globally no longer recommended for clinical use because of widespread resistance among circulating influenza A viruses (see below). By contrast, NA inhibitors target the enzymatic activity of the viral NA protein. Oseltamivir is delivered orally as the prodrug oseltamivir phosphate, which is converted to its active carboxylate form in the liver; zanamivir is inhaled as a powder (limiting its use in those with underlying respiratory problems); and peramivir is administered intravenously, which is important for patients who have been hospitalized 173 . All three drugs have received approval in the United States, Europe, Canada, Australia, Japan, Taiwan and Korea and act by mimicking the binding of sialic acid in the active site of NA on influenza A and influenza B viruses 174 .
Oseltamivir and zanamivir are effective for prophylaxis and post-exposure prophylaxis in individuals 175 . However, the randomized controlled trials of these drugs were conducted in patients with uncomplicated influenza; accordingly, observational data must be used to assess effectiveness in patients who are severely ill and have been hospitalized, in whom the need is arguably greater. Despite this limitation, study results consistently report improved outcomes associated with NA inhibitor use, including reduced risk of pneumonia and hospitalization, and reduced risk of mortality in patients who have been hospitalized 176 , 177 , 178 . Another consistent finding is that better results are obtained with early administration of NA inhibitors (within 2 days of symptom onset), although later administration can still be beneficial in severe cases.
The emergence of drug-resistant viruses is a major challenge for the antiviral field. Adamantane resistance (conferred by an S31N mutation in the RNA segment encoding M2) first emerged in influenza A H3N2 viruses in 2003 and became prevalent worldwide by 2008 (ref. 179 ). The pandemic 2009 influenza A H1N1 virus, which is presently circulating as a seasonal H1N1 virus, is also resistant to the adamantanes, as its M RNA segment (Fig. 1 ) was inherited from a virus that carried the S31N mutation 180 . Thus, since 2009, only the NA inhibitors have been able to provide protection, with the currently circulating human influenza A and influenza B viruses being generally sensitive to oseltamivir and zanamivir (Table 2 ). However, emergence of oseltamivir resistance is of particular concern for influenza A H1N1 viruses because it has happened in the past; in 2007, resistant influenza A H1N1 viruses began to circulate and quickly became dominant by the 2008–2009 season 181 . The resistant phenotype is associated with an H275Y mutation in the RNA segment encoding NA, and studies have shown that, in the background of other permissive mutations, H275Y-mutant viruses do not display any fitness deficits, which explains their ability to circulate so readily 182 , 183 . Of note, these viruses remain sensitive to zanamivir. Perhaps fortuitously, the oseltamivir-resistant seasonal influenza A H1N1 virus was displaced by the introduction of the oseltamivir-sensitive pandemic influenza A H1N1 virus in 2009. Since then, surveillance efforts have monitored NA inhibitor sensitivity closely and, although oseltamivir-resistant isolates of the 2009 influenza A H1N1 virus have been detected, these are primarily (but not exclusively) from patients undergoing therapy 184 . Susceptibility to NA inhibitor is assessed by measuring NA enzyme inhibition in a functional assay using cultured virus. Alternatively, RT-PCR capable of detecting single nucleotide polymorphisms (or RT-PCR followed by sequencing) is used to detect known resistance mutations in NA 185 , but the functional assay may be required if novel mutations are present.
There is a higher prevalence of resistant virus in children and individuals who are immunocompromised, which is believed to be related to higher viral loads and prolonged virus shedding in these individuals 186 , 187 . Also, patients who have been hospitalized are more likely to be treated for longer periods, which will increase pressure on the virus and the likelihood that a resistant strain will emerge. Although this outcome may be predictable, oseltamivir resistance arose in 2007 seemingly in the absence of drug pressure; given the correct background of secondary mutations, the same outcome is a possibility for the current influenza A H1N1 virus 188 . Oseltamivir resistance among influenza A H3N2 viruses and influenza B viruses has also been reported, albeit at a much lower frequency than for influenza A H1N1 (ref. 189 ).
The elimination of adamantanes as clinical therapy combined with the concerns over increasing oseltamivir resistance illustrates the need for new influenza antiviral drugs. Several candidates are now in clinical trial, including additional NA inhibitors that can be delivered intravenously, such as intravenous formulations of zanamivir. Another NA inhibitor, laninamivir, has been approved for use in Japan; this drug is inhaled and long-acting and requires less frequent administration than oseltamivir or zanamivir 190 . Ideally, future influenza therapies will involve a cocktail of drugs with different mechanisms that should increase the barrier to resistance. Thus, drugs other than NA inhibitors are being clinically tested; within this pipeline are favipiravir (which is a polymerase inhibitor for several RNA viruses 191 ), pimodivir (which prevents the cap binding required for viral RNA transcription 192 ), baloxavir marboxil (an inhibitor of the cap-dependent endonuclease activity of the influenza viral polymerase 193 ) and nitazoxanide (an inhibitor of HA maturation 194 ). Baloxavir marboxil received approval in Japan in 2018. Favipiravir has also been approved in Japan since 2014, but only for release from government stockpile in the event of a novel strain being unresponsive to current agents. Treatment of patients who have been hospitalized with immune plasma 195 or with hyperimmune immunoglobulin (pooled from plasma of donors with high titres of anti-influenza IgG) 196 is also being clinically evaluated. In addition, several monoclonal antibodies against the conserved region of the HA stalk are also under clinical development for the treatment of severe influenza infection 197 . Many more candidates are in preclinical development and will hopefully lead to improved options for influenza therapy in the future.
Non-pharmaceutical prevention strategies
In addition to vaccines and antiviral drugs, non-pharmaceutical interventions can help to slow the spread of influenza illness. These interventions include personal measures, such as hand washing and using alcohol-based sanitizers 198 , covering coughs and/or the nose and mouth when sick and staying at home when sick. Additionally, social distancing by closures of schools and places of gathering, quarantine measures and frequent cleaning of potentially virus-contaminated surfaces, such as door knobs, can also slow the spread. Mathematical modelling studies suggest that non-pharmaceutical interventions have a substantial effect on lowering the attack rate of pandemic influenza before vaccines are available 199 .
Management of acute influenza has traditionally relied on supportive measures such as control of fever, symptomatic treatment, rehydration and treatment of complications, such as bacterial pneumonia, if they occur. Supportive care is still the mainstay of management of uncomplicated influenza. However, as described above, several antiviral agents have been developed for influenza or are in active clinical development. Furthermore, given the natural animal reservoirs of influenza (Fig. 3 ), prevention of transmission from these reservoirs to humans is key. Water treatment, indoor raising and biosecurity are the primary management goals; these strategies are followed by quarantine, stamping out and the ultimate use of animal vaccination.
Human influenza
People with clinical symptoms of influenza virus infection should remain home and avoid contact with non-infected people until symptoms alleviate. As the level of virus replication is correlated with the clinical severity of illness, strategies to limit viral replication with antiviral agents would be expected to be effective in acute influenza illness. However, adults with an intact immune system who have had previous influenza infections rapidly limit the replication of these viruses without intervention, so the opportunity to attenuate viral replication with antiviral agents is limited. Effective use of antivirals for seasonal influenza in otherwise healthy adults requires early initiation of therapy, generally within the first 48 hours of symptom onset. However, decisions regarding treatment should consider the somewhat small benefit demonstrated in clinical trials and the overall low risk of complications 177 . The benefits of later therapy in otherwise uncomplicated influenza are unclear.
Patients with underlying immune defects, those with more-severe disease or those who have a higher risk of influenza complications 200 may benefit from antiviral administration, even if initiated after 48 hours of symptom onset 201 if they are still shedding virus. Antiviral therapy is strongly considered if the illness is progressing and symptoms are worsening, particularly if the patient continues to test positive for influenza virus. Indeed, prescription of antivirals for high-risk outpatients with acute respiratory illness is beneficial 202 . Furthermore, a diagnosis of influenza in patients who have been hospitalized with community-acquired pneumonia should be confirmed to increase antiviral treatment rates in patients 203 . Studies suggest that, since the 2009 pandemic, the use of antivirals in patients who have been hospitalized with influenza has increased 204 , which is an improvement. Complications of influenza virus infection might require specific treatments, as is the case with secondary bacterial pneumonia, which should be treated with appropriate antibiotics.
Finally, consideration should also be given to the possible use of immunotherapy aimed at controlling exacerbated pro-inflammatory responses in patients with severe symptoms, especially in combination with antiviral therapy. This approach is supported by recent clinical studies suggesting beneficial effects when inhibitors of cytokine production and leukocyte recruitment were added to NA inhibitor therapy 205 .
Animal influenza
As influenza in wild and domestic waterfowl is primarily spread through contaminated water, water treatment (with, for example, chlorine and ultraviolet light), biosecurity and indoor raising of animals are the main strategies to prevent transmission. Biosecurity includes placing poultry houses and piggeries on high ground away from high-density and backyard farms, live poultry markets and bodies of water to minimize contact with waterfowl and passerine birds. Risk assessment and training of personnel to change outdoor clothing, disinfect foot baths and treat food and water are also important aspects of biosecurity.
After a novel influenza virus has breached biosecurity, a strategy that includes rapid detection and stamping out is optimal 206 . Stamping out is easier when animals display signs of severe infection, but these can go unnoticed when LPAI infection occurs. After initial emergence of HPAI H5 or H7 in poultry, quarantine, culling, decontamination and compensation of farmers with restricted zones of movement are imposed. This classic strategy was successful in the majority of Eurasian countries to which HPAI H5N1 spread 206 . However, late detection and widespread dissemination of HPAI H5N1 in China, Indonesia, Vietnam, Bangladesh and Egypt resulted in endemicity, making virus eradication difficult 206 . One difficulty in eradication is inadequate provision of compensation to farmers, who will be incentivized to hide infected animals.
Poultry vaccines use inactivated whole virus in oil emulsion, recombinant DNA or vectorized vaccines. These vaccines can be highly effective at reducing disease signs and inter-species spread, but they do not induce sterilizing immunity and have not resulted in eradication from endemic countries. Poultry vaccination is highly contentious; both the United Nations Food and Agriculture Organization (FAO) and the World Organisation for Animal Health (OIE) state that long-term vaccination without additional measures is not recommended 207 . Although poultry vaccination dramatically reduced human cases of H5N1 in Vietnam in 2006, it did not eradicate the virus and likely contributed to the antigenic diversity. On the other hand, vaccination used in combination with unvaccinated sentinel chickens and stamping out can achieve control and local eradication 208 .
Live poultry markets have been recognized as a breeding ground of influenza viruses. Changes to live poultry marketing achieved by implementing so-called clean days (that is, days when stalls are cleared of unsold poultry and disinfected) and no carry-over (that is, no infectious material remains when the next live birds arrive after disinfection) of live poultry improved hygiene and reduced virus spread 209 , 210 . A definitive solution would be the closing of all live poultry markets. Indeed, market closure contributed to the reduction of the spread of HPAI H5N1 in Hong Kong and H7N9 transmissions to humans in China 211 . Such changes without public training risk poultry smuggling from unregulated sources.
The management of influenza in pigs or horses is dependent on the use of vaccines. In pigs, influenza is a minor disease problem for which biosecurity is paramount, and vaccination uses primarily whole inactivated virus in oil emulsion. In horses, the problem with vaccination is the short-term protection offered, but antigenically matched adjuvanted inactivated vaccines and LAIVs are efficacious 212 .
Quality of life
Uncomplicated influenza in otherwise healthy adults and children is rarely fatal but can be highly disruptive and lead to substantial absences from work or school. Recovery can be prolonged, with persistent fatigue and malaise that can last for several weeks after recovery from the other symptoms. Influenza is associated with decreased job performance in adults 213 and reduced levels of independent functioning in older adults 214 . As a result, the loss of productivity by affected individuals contributes substantially to the overall economic effect of influenza. Mild abnormalities in pulmonary function are frequently experienced by healthy adults and also can take several weeks to return to normal. In a 2007 study, direct yearly medical costs associated with influenza in the United States were estimated to be approximately $10.4 billion, whereas projected lost earnings owing to illness and loss of life were estimated at $16.3 billion 215 .
One of the unique features of influenza is the somewhat high frequency with which it is associated with pulmonary complications such as pneumonia, including primary influenza virus pneumonia and secondary bacterial pneumonia. However, during an outbreak of influenza, many patients do not clearly fit into either of these presentations and may instead have mixed viral and bacterial pneumonia. Primary influenza viral pneumonia was well documented in the 1957–1958 pandemic, although many deaths of young healthy adults in the 1918–1919 pandemic were also the result of primary pneumonia 216 . Primary pneumonia occurs predominantly among patients with influenza virus infection who also have underlying cardiovascular disease, especially rheumatic heart disease with mitral stenosis, but may occur in a variety of settings. Primary viral influenza pneumonia is characterized by a rapid progression of fever, cough, dyspnoea (laboured breathing) and cyanosis (low oxygen levels resulting in bluish skin), with chest radiographs often revealing bilateral findings consistent with acute respiratory distress syndrome.
The classic presentation of secondary bacterial pneumonia is that of typical influenza illness followed by a period of improvement, which is then followed by recurrent fever, cough, sputum production and consolidation (fluid build-up in the lungs). Indeed, bacterial co-infections were present at high frequency during the 1918 pandemic 217 , 218 . The two pathogens that are currently most commonly associated with influenza are Streptococcus pneumoniae and Staphylococcus aureus 219 . The mechanisms by which influenza infection uniquely predisposes to bacterial superinfection are being actively investigated. In addition to pneumonia, multiple other pulmonary complications of influenza are recognized, including bronchiolitis (inflammation of the bronchioles) and croup (laryngotracheobronchitis) and exacerbations of chronic bronchitis or asthma.
Non-respiratory complications have also been reported in association with acute influenza infection 220 . Myositis, with tender leg muscles and elevated serum creatine phosphokinase, has been reported primarily in children 221 . Both myocarditis and pericarditis have been rarely associated with influenza A or influenza B virus infection. Because influenza may predispose to superinfection with S. aureus , toxic-shock syndrome, a clinical illness characterized by hypotension, fever, erythroderma (reddening of the skin), desquamation (skin peeling) and multisystem involvement, has also been observed following influenza, primarily in children 222 . Guillain–Barré syndrome has been reported to occur after influenza A infection, as it has after numerous other infections, but no definite aetiological relationship has been established. In addition, cases of transverse myelitis (inflammation and myelin loss around the spinal cord) and encephalopathy have occurred rarely in association with influenza 223 . An epidemic of an atypical form of encephalitis known as encephalitis lethargica (characterized by headache, drowsiness and coma) spread around the world after the 1918 influenza pandemic and then practically disappeared in 1930, suggesting that this was a sequela caused by the pandemic 224 . However, as acute influenza is a common disease event, a temporal association with other disease entities does not always indicate a cause and effect relationship.
A better understanding of the ecological, viral and host determinants permitting inter-species transmission and adaptation of influenza A viruses to new hosts may facilitate earlier recognition and evidence-based risk assessment of influenza A viruses that may threaten either animal or human health. Better understanding will also provide the basis for implementing interventions aimed at preventing emergence at the source. For example, the understanding that domestic ducks serve as an interface between the gene pools of wild aquatic birds and terrestrial poultry supports the idea that measures to reduce exposure of domestic ducks to wild birds are warranted and the idea that separation of aquatic and terrestrial poultry through wholesale and retail live poultry marketing chains in Asia is also valid.
Despite the availability of vaccines and antiviral agents, influenza continues to be the most important cause of viral respiratory disease associated with human hospitalizations and deaths. We should not accept such a burden for, in theory, a vaccine-preventable disease. The current seasonal human influenza vaccine necessitates annual administration, is not optimal and varies in effectiveness from year to year. Pandemic years and seasonal years when the vaccine is mismatched are especially concerning. Mismatches in vaccine occur not only when the most prevalent antigenic strains are missed but also when the vaccine becomes antigenically mismatched owing to adaptations to egg culture. For example, the 2016–2017 seasonal influenza vaccine produced in eggs lacked a glycosylation site present in the influenza A H3N2 circulating strains. This change was due to egg adaptation and led to an alteration of the antigenicity of the influenza vaccine component 225 . Tissue culture to manufacture influenza virus vaccines might be less prone to HA adaptations, but it does not completely prevent them. In this respect, the aforementioned recombinant baculovirus-produced influenza vaccines have an advantage in that they do not select for HA adaptive mutations.
The management of both animal and human influenza by vaccination is less than optimal. The development of a highly efficacious universal vaccine and genetically resistant animals (that have been engineered or that have evolved to be so) are future options. Even the development of new vaccines that provide more-durable and more-effective protection against circulating viruses would represent major improvements. An exhaustive exploration in humans of adjuvants, live attenuated approaches, vaccine platforms and antigen design is required to identify the best approaches to improve immunogenicity without compromising safety. Although clinical studies to demonstrate influenza vaccine efficacy are costly and challenging, it is imperative that we apply modern science and technology to improve the existing influenza virus vaccines. In this respect, more studies are needed to improve our understanding of the correlates of immune protection in humans and to develop immunological, molecular and genetic tools for the better use of animal models of human influenza. Moreover, although experimental human challenges have been developed to quantify protective responses in patients, these are not yet optimal, as in general they require infection protocols that do not mimic natural infections 226 . The same principles apply to the development of new influenza antiviral agents. Finally, a better understanding of the risk factors, both in the host as well as in the virus, that predispose to more-severe influenza is also needed.
One hundred years after the devastating 1918 influenza pandemic, we still are not able to optimally prevent and treat influenza. However, substantial scientific advances have been made, such that our understanding of the molecular biology, immunology, host response, tropism and pathophysiology of influenza has improved, as has our understanding of the influenza virus itself. We hope that in the next decade, productive partnerships will be established between government, philanthropic agencies, academia and industry to facilitate more scientific discoveries and that these discoveries will translate to improvements in treatment and prevention of influenza. In this respect, the US National Institute of Allergy and Infectious Diseases is, for example, launching new research initiatives and strengthening existing research programmes to support inter-collaborative research to accelerate the development of improved influenza vaccines that provide durable protection from seasonal and pandemic influenza virus strains 227 — a promising step towards the generation of a universal influenza vaccine. Likely, the induction in the respiratory mucosa of effective and durable antibody responses against conserved conformational epitopes in viral HA and NA and T cell responses against conserved viral protein epitopes representing the diversity of human HLA will be required for the development of such a universal influenza virus vaccine.
Sellers, S. A., Hagan, R. S., Hayden, F. G. & Fischer, W. A. 2nd. The hidden burden of influenza: A review of the extra-pulmonary complications of influenza infection. Influenza Other Respir. Viruses 11 , 372–393 (2017).
PubMed PubMed Central Google Scholar
Kwong, J. C. et al. Acute myocardial infarction after laboratory-confirmed influenza infection. N. Engl. J. Med. 378 , 345–353 (2018).
PubMed Google Scholar
Matsuzaki, Y. et al. Clinical features of influenza C virus infection in children. J. Infect. Dis. 193 , 1229–1235 (2006).
Olsen, B. et al. Global patterns of influenza a virus in wild birds. Science 312 , 384–388 (2006).
CAS PubMed Google Scholar
Webster, R. G., Bean, W. J., Gorman, O. T., Chambers, T. M. & Kawaoka, Y. Evolution and ecology of influenza A viruses. Microbiol. Rev. 56 , 152–179 (1992).
CAS PubMed PubMed Central Google Scholar
Tong, S. et al. A distinct lineage of influenza A virus from bats. Proc. Natl Acad. Sci. USA 109 , 4269–4274 (2012).
Ma, W., García-Sastre, A. & Schwemmle, M. Expected and unexpected features of the newly discovered bat influenza A-like viruses. PLOS Pathog. 11 , e1004819 (2015).
Google Scholar
Fouchier, R. A. et al. Characterization of a novel influenza A virus hemagglutinin subtype (H16) obtained from black-headed gulls. J. Virol. 79 , 2814–2822 (2005).
Osterhaus, A. D., Rimmelzwaan, G. F., Martina, B. E., Bestebroer, T. M. & Fouchier, R. A. Influenza B virus in seals. Science 288 , 1051–1053 (2000).
Guo, Y. J., Jin, F. G., Wang, P., Wang, M. & Zhu, J. M. Isolation of influenza C virus from pigs and experimental infection of pigs with influenza C virus. J. Gen. Virol. 64 , 177–182 (1983).
Hause, B. M. et al. Isolation of a novel swine influenza virus from Oklahoma in 2011 which is distantly related to human influenza C viruses. PLOS Pathog. 9 , e1003176 (2013).
Hause, B. M. et al. Characterization of a novel influenza virus in cattle and Swine: proposal for a new genus in the Orthomyxoviridae family. MBio 5 , e00031–00014 (2014).
Smith, G. J. et al. Origins and evolutionary genomics of the 2009 swine-origin H1N1 influenza A epidemic. Nature 459 , 1122–1125 (2009).
Mena, I. et al. Origins of the 2009 H1N1 influenza pandemic in swine in Mexico. elife 5 , e.16777 (2016). This reference tracks the origins of a human pandemic influenza A virus for the first time .
Hayden, F. Developing new antiviral agents for influenza treatment: what does the future hold? Clin. Infect. Dis. 48 (Suppl. 1), S3–S13 (2009).
Hurt, A. C. et al. Antiviral resistance during the 2009 influenza A H1N1 pandemic: public health, laboratory, and clinical perspectives. Lancet Infect. Dis. 12 , 240–248 (2012).
Rambaut, A. et al. The genomic and epidemiological dynamics of human influenza A virus. Nature 453 , 615–619 (2008).
Russell, C. A. et al. The global circulation of seasonal influenza A (H3N2) viruses. Science 320 , 340–346 (2008). Together with reference 17, this seminal study proposes intriguing models of how influenza viruses are maintained in the human population and transmitted globally during seasonal epidemics .
Bahl, J. et al. Temporally structured metapopulation dynamics and persistence of influenza A H3N2 virus in humans. Proc. Natl Acad. Sci. USA 108 , 19359–19364 (2011).
Yu, H. et al. Characterization of regional influenza seasonality patterns in China and implications for vaccination strategies: spatio-temporal modeling of surveillance data. PLOS Med. 10 , e1001552 (2013).
Centers for Disease Control and Prevention. Estimated influenza illnesses and hospitalizations averted by vaccination — United States, 2014–15 influenza season. CDC https://www.cdc.gov/flu/about/disease/2014-15.htm (2015).
Cohen, S. A., Chui, K. K. & Naumova, E. N. Influenza vaccination in young children reduces influenza-associated hospitalizations in older adults, 2002–2006. J. Am. Geriatr. Soc. 59 , 327–332 (2011).
Gostic, K. M., Ambrose, M., Worobey, M. & Lloyd-Smith, J. O. Potent protection against H5N1 and H7N9 influenza via childhood hemagglutinin imprinting. Science 354 , 722–726 (2016).
Lozano, R. et al. Global and regional mortality from 235 causes of death for 20 age groups in 1990 and 2010: a systematic analysis for the Global Burden of Disease Study 2010. Lancet 380 , 2095–2128 (2012).
Iuliano, A. D. et al. Estimates of global seasonal influenza-associated respiratory mortality: a modelling study. Lancet 391 , 1285–1300 (2018).
Thompson, W. W. et al. Mortality associated with influenza and respiratory syncytial virus in the United States. JAMA 289 , 179–186 (2003). This study represents a classic analysis of hospital-acquired pneumonia and influenza deaths that establishes the impact of seasonal influenza in the United States .
Flannery, B. et al. Influenza vaccine effectiveness against pediatric deaths: 2010–2014. Pediatrics 139 , https://doi.org/10.1542/peds.2016-4244 (2017).
Thompson, W. W. et al. Influenza-associated hospitalizations in the United States. JAMA 292 , 1333–1340 (2004).
Walsh, E. E., Cox, C. & Falsey, A. R. Clinical features of influenza A virus infection in older hospitalized persons. J. Am. Geriatr. Soc. 50 , 1498–1503 (2002).
Jain, S. et al. Hospitalized patients with 2009 H1N1 influenza in the United States, April-June 2009. N. Engl. J. Med. 361 , 1935–1944 (2009).
Keren, R. et al. Neurological and neuromuscular disease as a risk factor for respiratory failure in children hospitalized with influenza infection. JAMA 294 , 2188–2194 (2005).
Neuzil, K. M., Reed, G. W., Mitchel, E. F., Simonsen, L. & Griffin, M. R. Impact of influenza on acute cardiopulmonary hospitalizations in pregnant women. Am. J. Epidemiol. 148 , 1094–1102 (1998).
Van Kerkhove, M. D. et al. Risk factors for severe outcomes following 2009 influenza A (H1N1) infection: a global pooled analysis. PLOS Med. 8 , e1001053 (2011).
Karlsson, E. A. et al. A perfect storm: Increased colonization and failure of vaccination leads to severe secondary bacterial infection in influenza virus-infected obese mice. MBio 8 , e00889-17 (2017).
Ciancanelli, M. J. et al. Life-threatening influenza and impaired interferon amplification in human IRF7 deficiency. Science 348 , 448–453 (2015).
Everitt, A. R. et al. IFITM3 restricts the morbidity and mortality associated with influenza. Nature 484 , 519–523 (2012).
Allen, E. K. et al. SNP-mediated disruption of CTCF binding at the IFITM3 promoter is associated with risk of severe influenza in humans. Nat. Med. 23 , 975–983 (2017).
van Gils, J. A. et al. Hampered foraging and migratory performance in swans infected with low-pathogenic avian influenza A virus. PLOS ONE 2 , e184 (2007).
Guan, Y. & Smith, G. J. The emergence and diversification of panzootic H5N1 influenza viruses. Virus Res. 178 , 35–43 (2013).
Ip, H. S. et al. Novel Eurasian highly pathogenic avian influenza A H5 viruses in wild birds, WA, USA, 2014. Emerg. Infect. Dis. 21 , 886–890 (2015).
Ke, C. et al. Human infection with highly pathogenic avian influenza A(H7N9) virus. China. Emerg. Infect. Dis. 23 , 1332–1340 (2017).
Lee, C. T. et al. Outbreak of influenza A(H7N2) among cats in an animal shelter with cat-to-human transmission-New York City, 2016. Clin. Infect. Dis. 65 , 1927–1929 (2017).
Fouchier, R. A. et al. Avian influenza A virus (H7N7) associated with human conjunctivitis and a fatal case of acute respiratory distress syndrome. Proc. Natl Acad. Sci. USA 101 , 1356–1361 (2004).
Perkins, L. E. & Swayne, D. E. Pathogenicity of a Hong Kong-origin H5N1 highly pathogenic avian influenza virus for emus, geese, ducks, and pigeons. Avian Dis. 46 , 53–63 (2002).
Hulse-Post, D. J. et al. Role of domestic ducks in the propagation and biological evolution of highly pathogenic H5N1 influenza viruses in Asia. Proc. Natl Acad. Sci. USA 102 , 10682–10687 (2005).
Fournie, G. et al. Interventions for avian influenza A (H5N1) risk management in live bird market networks. Proc. Natl Acad. Sci. USA 110 , 9177–9182 (2013).
Vincent, A. et al. Review of influenza A virus in swine worldwide: a call for increased surveillance and research. Zoonoses Publ. Health 61 , 4–17 (2014).
CAS Google Scholar
Neumann, G. & Kawaoka, Y. The first influenza pandemic of the new millennium. Influenza Other Respir. Viruses 5 , 157–166 (2011).
Jhung, M. A. et al. Outbreak of variant influenza A(H3N2) virus in the United States. Clin. Infect. Dis. 57 , 1703–1712 (2013).
Crawford, P. C. et al. Transmission of equine influenza virus to dogs. Science 310 , 482–485 (2005).
Li, S. et al. Avian-origin H3N2 canine influenza A viruses in Southern China. Infect. Genet. Evol. 10 , 1286–1288 (2010).
Yamada, S. et al. Haemagglutinin mutations responsible for the binding of H5N1 influenza A viruses to human-type receptors. Nature 444 , 378–382 (2006).
van Riel, D. et al. H5N1 virus attachment to lower respiratory tract. Science 312 , 399 (2006).
Hirst, G. K. Studies of antigenic differences among strains of influenza A by means of red cell agglutination. J. Exp. Med. 78 , 407–423 (1943).
Barr, I. G. et al. WHO recommendations for the viruses used in the 2013–2014 Northern Hemisphere influenza vaccine: epidemiology, antigenic and genetic characteristics of influenza A(H1N1)pdm09, A(H3N2) and B influenza viruses collected from October 2012 to January 2013. Vaccine 32 , 4713–4725 (2014).
Sandbulte, M. R. et al. Discordant antigenic drift of neuraminidase and hemagglutinin in H1N1 and H3N2 influenza viruses. Proc. Natl Acad. Sci. USA 108 , 20748–20753 (2011).
Kilbourne, E. D., Laver, W. G., Schulman, J. L. & Webster, R. G. Antiviral activity of antiserum specific for an influenza virus neuraminidase. J. Virol. 2 , 281–288 (1968).
Couzens, L. et al. An optimized enzyme-linked lectin assay to measure influenza A virus neuraminidase inhibition antibody titers in human sera. J. Virol. Methods 210 , 7–14 (2014).
Voeten, J. T. et al. Antigenic drift in the influenza A virus (H3N2) nucleoprotein and escape from recognition by cytotoxic T lymphocytes. J. Virol. 74 , 6800–6807 (2000).
Smith, D. J. et al. Mapping the antigenic and genetic evolution of influenza virus. Science 305 , 371–376 (2004). This study describes the concept of antigenic cartography and uses antigenic cartography for the visualization and quantification of antigenic drift of human H3N2 influenza A viruses over 35 years .
Koel, B. F. et al. Substitutions near the receptor binding site determine major antigenic change during influenza virus evolution. Science 342 , 976–979 (2013).
Koel, B. F. et al. Antigenic variation of clade 2.1 H5N1 virus is determined by a few amino acid substitutions immediately adjacent to the receptor binding site. MBio 5 , e01070-01014 (2014).
Lewis, N. S. et al. Antigenic and genetic evolution of equine influenza A (H3N8) virus from 1968 to 2007. J. Virol. 85 , 12742–12749 (2011).
de Jong, J. C. et al. Antigenic and genetic evolution of swine influenza A (H3N2) viruses in Europe. J. Virol. 81 , 4315–4322 (2007).
Kendal, A. P., Noble, G. R., Skehel, J. J. & Dowdle, W. R. Antigenic similarity of influenza A (H1N1) viruses from epidemics in 1977—1978 to “Scandinavian” strains isolated in epidemics of 1950–1951. Virology 89 , 632–636 (1978).
Herfst, S. et al. Airborne transmission of influenza A/H5N1 virus between ferrets. Science 336 , 1534–1541 (2012).
Imai, M. et al. Experimental adaptation of an influenza H5 HA confers respiratory droplet transmission to a reassortant H5 HA/H1N1 virus in ferrets. Nature 486 , 420–428 (2012).
Russell, C. A. et al. The potential for respiratory droplet-transmissible A/H5N1 influenza virus to evolve in a mammalian host. Science 336 , 1541–1547 (2012).
Yoon, S. W., Webby, R. J. & Webster, R. G. Evolution and ecology of influenza A viruses. Curr. Top. Microbiol. Immunol. 385 , 359–375 (2014).
Webster, R. G. & Hulse, D. J. Microbial adaptation and change: avian influenza. Rev. Sci. Tech. 23 , 453–465 (2004).
Andino, R. & Domingo, E. Viral quasispecies. Virology 479–480 , 46–51 (2015).
Tumpey, T. M. et al. A two-amino acid change in the hemagglutinin of the 1918 influenza virus abolishes transmission. Science 315 , 655–659 (2007). This study provides the first evidence that the receptor specificity of the HA of influenza A virus plays a major role in the respiratory transmission of these viruses .
Mitnaul, L. J. et al. Balanced hemagglutinin and neuraminidase activities are critical for efficient replication of influenza A virus. J. Virol. 74 , 6015–6020 (2000).
Reed, M. L. et al. The pH of activation of the hemagglutinin protein regulates H5N1 influenza virus pathogenicity and transmissibility in ducks. J. Virol. 84 , 1527–1535 (2010).
Hatta, M., Gao, P., Halfmann, P. & Kawaoka, Y. Molecular basis for high virulence of Hong Kong H5N1 influenza A viruses. Science 293 , 1840–1842 (2001).
Mehle, A. & Doudna, J. A. Adaptive strategies of the influenza virus polymerase for replication in humans. Proc. Natl Acad. Sci. USA 106 , 21312–21316 (2009).
Riegger, D. et al. The nucleoprotein of newly emerged H7N9 influenza A virus harbors a unique motif conferring resistance to antiviral human MxA. J. Virol. 89 , 2241–2252 (2015).
Campbell, P. J. et al. The M segment of the 2009 pandemic influenza virus confers increased neuraminidase activity, filamentous morphology, and efficient contact transmissibility to A/Puerto Rico/8/1934-based reassortant viruses. J. Virol. 88 , 3802–3814 (2014).
Medina, R. A. & García-Sastre, A. Influenza A viruses: new research developments. Nat. Rev. Microbiol. 9 , 590–603 (2011).
Tong, S. et al. New world bats harbor diverse influenza A viruses. PLOS Pathog. 9 , e1003657 (2013).
Moreira, E. A. et al. Synthetically derived bat influenza A-like viruses reveal a cell type- but not species-specific tropism. Proc. Natl Acad. Sci. USA 113 , 12797–12802 (2016).
Mibayashi, M. et al. Inhibition of retinoic acid-inducible gene I-mediated induction of beta interferon by the NS1 protein of influenza A virus. J. Virol. 81 , 514–524 (2007).
Pichlmair, A. et al. RIG-I-mediated antiviral responses to single-stranded RNA bearing 5′-phosphates. Science 314 , 997–1001 (2006).
Rajsbaum, R. et al. Species-specific inhibition of RIG-I ubiquitination and IFN induction by the influenza A virus NS1 protein. PLOS Pathog. 8 , e1003059 (2012).
Nemeroff, M. E., Barabino, S. M., Li, Y., Keller, W. & Krug, R. M. Influenza virus NS1 protein interacts with the cellular 30 kDa subunit of CPSF and inhibits 3′end formation of cellular pre-mRNAs. Mol. Cell 1 , 991–1000 (1998).
Satterly, N. et al. Influenza virus targets the mRNA export machinery and the nuclear pore complex. Proc. Natl Acad. Sci. USA 104 , 1853–1858 (2007).
Marazzi, I. et al. Suppression of the antiviral response by an influenza histone mimic. Nature 483 , 428–433 (2012).
Chauche, C. et al. Mammalian adaptation of an avian influenza A virus involves stepwise changes in NS1. J. Virol. e01875-17 (2017).
Li, S., Min, J. Y., Krug, R. M. & Sen, G. C. Binding of the influenza A virus NS1 protein to PKR mediates the inhibition of its activation by either PACT or double-stranded RNA. Virology 349 , 13–21 (2006).
Min, J. Y. & Krug, R. M. The primary function of RNA binding by the influenza A virus NS1 protein in infected cells: Inhibiting the 2′-5′ oligo (A) synthetase/RNase L pathway. Proc. Natl Acad. Sci. USA 103 , 7100–7105 (2006).
Conenello, G. M. & Palese, P. Influenza A virus PB1-F2: a small protein with a big punch. Cell Host Microbe 2 , 207–209 (2007).
Graef, K. M. et al. The PB2 subunit of the influenza virus RNA polymerase affects virulence by interacting with the mitochondrial antiviral signaling protein and inhibiting expression of beta interferon. J. Virol. 84 , 8433–8445 (2010).
Jagger, B. W. et al. An overlapping protein-coding region in influenza A virus segment 3 modulates the host response. Science 337 , 199–204 (2012).
Crotta, S. et al. Type I and type III interferons drive redundant amplification loops to induce a transcriptional signature in influenza-infected airway epithelia. PLOS Pathog. 9 , e1003773 (2013).
Helft, J. et al. Cross-presenting CD103+ dendritic cells are protected from influenza virus infection. J. Clin. Invest. 122 , 4037–4047 (2012).
Zhu, L. et al. High level of neutrophil extracellular traps correlates with poor prognosis of severe influenza A infection. J. Infect. Dis. 217 , 428–437 (2018).
Walsh, K. B. et al. Suppression of cytokine storm with a sphingosine analog provides protection against pathogenic influenza virus. Proc. Natl Acad. Sci. USA 108 , 12018–12023 (2011).
Sridhar, S. et al. Cellular immune correlates of protection against symptomatic pandemic influenza. Nat. Med. 19 , 1305–1312 (2013).
Wilkinson, T. M. et al. Preexisting influenza-specific CD4+ T cells correlate with disease protection against influenza challenge in humans. Nat. Med. 18 , 274–280 (2012).
Quinones-Parra, S. et al. Preexisting CD8+ T cell immunity to the H7N9 influenza A virus varies across ethnicities. Proc. Natl Acad. Sci. USA 111 , 1049–1054 (2014).
Wang, Z. et al. Recovery from severe H7N9 disease is associated with diverse response mechanisms dominated by CD8 + T cells. Nat. Commun. 6 , 6833 (2015). This manuscript provides evidence that early cross-reactive CD8 + T cell immunity plays an important part in recovery from severe avian influenza A H7N9 virus-induced disease .
van de Sandt, C. E. et al. Human influenza A virus-specific CD8+ T cell response is long-lived. J. Infect. Dis. 212 , 81–85 (2015).
Sun, J. C. & Bevan, M. J. Defective CD8 T cell memory following acute infection without CD4 T cell help. Science 300 , 339–342 (2003).
McKinstry, K. K. et al. Memory CD4+ T cells protect against influenza through multiple synergizing mechanisms. J. Clin. Invest. 122 , 2847–2856 (2012).
Deliyannis, G. et al. Intranasal lipopeptide primes lung-resident memory CD8+ T cells for long-term pulmonary protection against influenza. Eur. J. Immunol. 36 , 770–778 (2006).
Pejoski, D., Zeng, W., Rockman, S., Brown, L. E. & Jackson, D. C. A lipopeptide based on the M2 and HA proteins of influenza A viruses induces protective antibody. Immunol. Cell. Biol. 88 , 605–611 (2010).
McMichael, A. J., Gotch, F. M., Noble, G. R. & Beare, P. A. Cytotoxic T cell immunity to influenza. N. Engl. J. Med. 309 , 13–17 (1983).
Topham, D. J., Tripp, R. A. & Doherty, P. C. CD8+ T cells clear influenza virus by perforin or Fas-dependent processes. J. Immunol. 159 , 5197–5200 (1997).
Doherty, P. C., Turner, S. J., Webby, R. G. & Thomas, P. G. Influenza and the challenge for immunology. Nat. Immunol. 7 , 449–455 (2006).
Sridhar, S., Brokstad, K. A. & Cox, R. J. Influenza vaccination strategies: comparing inactivated and live attenuated influenza vaccines. Vaccines 3 , 373–389 (2015).
Russ, B. E. et al. Distinct epigenetic signatures delineate transcriptional programs during virus-specific CD8 + T cell differentiation. Immunity 41 , 853–865 (2014).
Valkenburg, S. A. et al. Early priming minimizes the age-related immune compromise of CD8 + T cell diversity and function. PLOS Pathog. 8 , e1002544 (2012).
Smith, W., Andrewes, C. H. & Laidlaw, P. P. A virus obtained from influenza patients. Lancet 222 , 66–68 (1933). This study describes the first isolation of influenza virus. Importantly, it includes proof of protection from influenza virus infection by passive transfer of antibodies in the ferret model of influenza .
Wrammert, J. et al. Rapid cloning of high-affinity human monoclonal antibodies against influenza virus. Nature 453 , 667–671 (2008).
Gerhard, W., Yewdell, J., Frankel, M. E. & Webster, R. Antigenic structure of influenza virus haemagglutinin defined by hybridoma antibodies. Nature 290 , 713–717 (1981).
Angeletti, D. et al. Defining B cell immunodominance to viruses. Nat. Immunol. 18 , 456–463 (2017).
Andrews, S. F. et al. Immune history profoundly affects broadly protective B cell responses to influenza. Sci. Transl Med. 7 , 316ra192 (2015).
Hobson, D., Curry, R. L., Beare, A. S. & Ward-Gardner, A. The role of serum haemagglutination-inhibiting antibody in protection against challenge infection with influenza A2 and B viruses. J. Hyg. 70 , 767–777 (1972).
Yu, X. et al. Neutralizing antibodies derived from the B cells of 1918 influenza pandemic survivors. Nature 455 , 532–536 (2008).
Novel Swine-Origin Influenza A Virus Investigation Team et al. Emergence of a novel swine-origin influenza A (H1N1) virus in humans. N. Engl. J. Med. 360 , 2605–2615 (2009).
Manicassamy, B. et al. Protection of mice against lethal challenge with 2009 H1N1 influenza A virus by 1918-like and classical swine H1N1 based vaccines. PLOS Pathog. 6 , e1000745 (2010).
Steens, A. et al. Age-dependent patterns of infection and severity explaining the low impact of 2009 influenza A (H1N1): evidence from serial serologic surveys in the Netherlands. Am. J. Epidemiol. 174 , 1307–1315 (2011).
Heaton, N. S., Sachs, D., Chen, C. J., Hai, R. & Palese, P. Genome-wide mutagenesis of influenza virus reveals unique plasticity of the hemagglutinin and NS1 proteins. Proc. Natl Acad. Sci. USA 110 , 20248–20253 (2013).
Doud, M. B. & Bloom, J. D. Accurate measurement of the effects of all amino-acid mutations on influenza hemagglutinin. Viruses 8 , 155 (2016).
PubMed Central Google Scholar
Monto, A. S., Malosh, R. E., Petrie, J. G. & Martin, E. T. The doctrine of original antigenic sin: separating good from evil. J. Infect. Dis. 215 , 1782–1788 (2017).
Henry, C., Palm, A. E., Krammer, F. & Wilson, P. C. From original antigenic sin to the universal influenza virus vaccine. Trends Immunol. 39 , 70–79 (2017).
Linderman, S. L. et al. Potential antigenic explanation for atypical H1N1 infections among middle-aged adults during the 2013–2014 influenza season. Proc. Natl Acad. Sci. USA 111 , 15798–15803 (2014).
Li, Y. et al. Immune history shapes specificity of pandemic H1N1 influenza antibody responses. J. Exp. Med. 210 , 1493–1500 (2013).
Krammer, F. & Palese, P. Influenza virus hemagglutinin stalk-based antibodies and vaccines. Curr. Opin. Virol. 3 , 521–530 (2013).
Ekiert, D. C. & Wilson, I. A. Broadly neutralizing antibodies against influenza virus and prospects for universal therapies. Curr. Opin. Virol. 2 , 134–141 (2012).
Neirynck, S. et al. A universal influenza A vaccine based on the extracellular domain of the M2 protein. Nat. Med. 5 , 1157–1163 (1999).
Wohlbold, T. J. et al. Broadly protective murine monoclonal antibodies against influenza B virus target highly conserved neuraminidase epitopes. Nat. Microbiol. 2 , 1415–1424 (2017).
Wan, H. et al. Molecular basis for broad neuraminidase immunity: conserved epitopes in seasonal and pandemic H1N1 as well as H5N1 influenza viruses. J. Virol. 87 , 9290–9300 (2013).
Rajendran, M. et al. Analysis of anti-influenza virus neuraminidase antibodies in children, adults, and the elderly by ELISA and enzyme inhibition: evidence for original antigenic sin. MBio 8 , https://doi.org/10.1128/mBio.02281-16 (2017).
Wohlbold, T. J. & Krammer, F. In the shadow of hemagglutinin: a growing interest in influenza viral neuraminidase and its role as a vaccine antigen. Viruses 6 , 2465–2494 (2014).
DiLillo, D. J., Palese, P., Wilson, P. C. & Ravetch, J. V. Broadly neutralizing anti-influenza antibodies require Fc receptor engagement for in vivo protection. J. Clin. Invest. 126 , 605–610 (2016). This study demonstrates that immune responses induced against the conserved influenza virus HA stalk domain include broadly protective antibodies that activate effector cells via Fc–FcR interactions.
Jegaskanda, S. et al. Cross-reactive influenza-specific antibody-dependent cellular cytotoxicity antibodies in the absence of neutralizing antibodies. J. Immunol. 190 , 1837–1848 (2013).
Jegaskanda, S., Weinfurter, J. T., Friedrich, T. C. & Kent, S. J. Antibody-dependent cellular cytotoxicity is associated with control of pandemic H1N1 influenza virus infection of macaques. J. Virol. 87 , 5512–5522 (2013).
Seibert, C. W. et al. Recombinant IgA is sufficient to prevent influenza virus transmission in guinea pigs. J. Virol. 87 , 7793–7804 (2013).
Lowen, A. C. et al. Blocking interhost transmission of influenza virus by vaccination in the guinea pig model. J. Virol. 83 , 2803–2818 (2009).
Ohmit, S. E. & Monto, A. S. Symptomatic predictors of influenza virus positivity in children during the influenza season. Clin. Infect. Dis. 43 , 564–568 (2006).
Kumar, S. & Henrickson, K. J. Update on influenza diagnostics: lessons from the novel H1N1 influenza A pandemic. Clin. Microbiol. Rev. 25 , 344–361 (2012).
Petric, M., Comanor, L. & Petti, C. A. Role of the laboratory in diagnosis of influenza during seasonal epidemics and potential pandemics. J. Infect. Dis. 194 (Suppl. 2), S98–S110, https://doi.org/10.1086/507554 (2006).
Newton, D. W., Treanor, J. J. & Menegus, M. A. Clinical and laboratory diagnosis of influenza virus infections. Am. J. Manag. Care 6 , S265–S275 (2000).
Dunn, J. J., Woolstenhulme, R. D., Langer, J. & Carroll, K. C. Sensitivity of respiratory virus culture when screening with R-mix fresh cells. J. Clin. Microbiol. 42 , 79–82 (2004).
Nie, S. et al. Evaluation of Alere i Influenza A&B for rapid detection of influenza viruses A and B. J. Clin. Microbiol. 52 , 3339–3344 (2014).
Merckx, J. et al. Diagnostic accuracy of novel and traditional rapid tests for influenza infection compared with reverse transcriptase polymerase chain reaction: A systematic review and meta-analysis. Ann. Intern. Med. 167 , 394–409 (2017).
Bhattacharya, S. et al. Transcriptomic biomarkers to discriminate bacterial from nonbacterial infection in adults hospitalized with respiratory illness. Sci. Rep. 7 , 6548 (2017).
Salk, J. E. & Suriano, P. C. Importance of antigenic composition of influenza virus vaccine in protecting against the natural disease; observations during the winter of 1947–1948. Am. J. Publ. Health Nat. Health 39 , 345–355 (1949).
Plotkin, S. A., Orenstein, W. & Offit, P. (eds), Vaccines 6 th edn (Saunders, 2012)
Rudenko, L., Yeolekar, L., Kiseleva, I. & Isakova-Sivak, I. Development and approval of live attenuated influenza vaccines based on Russian master donor viruses: Process challenges and success stories. Vaccine 34 , 5436–5441 (2016).
Caspard, H., Mallory, R. M., Yu, J. & Ambrose, C. S. Live-attenuated influenza vaccine effectiveness in children from 2009 to 2015-2016: A systematic review and meta-analysis. Open Forum Infect. Dis. 4 , ofx111 (2017).
Manini, I. et al. Egg-independent influenza vaccines and vaccine candidates. Vaccines 5 , 18 (2017).
Centers for Disease Control and Prevention. Flublok seasonal influenza (flu) vaccine. CDC https://www.cdc.gov/flu/protect/vaccine/qa_flublok-vaccine.htm (2017).
Clark, T. W. et al. Trial of 2009 influenza A (H1N1) monovalent MF59-adjuvanted vaccine. N. Engl. J. Med. 361 , 2424–2435 (2009).
Nohynek, H. et al. AS03 adjuvanted AH1N1 vaccine associated with an abrupt increase in the incidence of childhood narcolepsy in Finland. PLOS ONE 7 , e33536 (2012).
DiazGranados, C. A. et al. Efficacy of high-dose versus standard-dose influenza vaccine in older adults. N. Engl. J. Med. 371 , 635–645 (2014).
Enami, M., Luytjes, W., Krystal, M. & Palese, P. Introduction of site-specific mutations into the genome of influenza virus. Proc. Natl Acad. Sci. USA 87 , 3802–3805 (1990).
Krammer, F. & Palese, P. Advances in the development of influenza virus vaccines. Nat. Rev. Drug Discov. 14 , 167–182 (2015).
Paules, C. I., Marston, H. D., Eisinger, R. W., Baltimore, D. & Fauci, A. S. The pathway to a universal influenza vaccine. Immunity 47 , 599–603 (2017).
Committee On Infectious Diseases. Recommendations for prevention and control of influenza in children, 2017–2018. Pediatrics 140 , e20172550 (2017).
Palese, P. & Wang, T. T. H5N1 influenza viruses: facts, not fear. Proc. Natl Acad. Sci. USA 109 , 2211–2213 (2012).
Gomaa, M. R. et al. Avian influenza A(H5N1) and A(H9N2) seroprevalence and risk factors for infection among Egyptians: a prospective, controlled seroepidemiological study. J. Infect. Dis. 211 , 1399–1407 (2015).
Wang, T. T., Parides, M. K. & Palese, P. Seroevidence for H5N1 influenza infections in humans: meta-analysis. Science 335 , 1463 (2012).
Varble, A. et al. Influenza A virus transmission bottlenecks are defined by infection route and recipient host. Cell Host Microbe 16 , 691–700 (2014).
Sobel Leonard, A., Weissman, D. B., Greenbaum, B., Ghedin, E. & Koelle, K. Transmission bottleneck size estimation from pathogen deep-sequencing data, with an application to human influenza A virus. J. Virol. 91 , e00171-17 (2017).
Osterholm, M. T., Kelley, N. S., Sommer, A. & Belongia, E. A. Efficacy and effectiveness of influenza vaccines: a systematic review and meta-analysis. Lancet Infect. Dis. 12 , 36–44 (2012).
DiazGranados, C. A., Denis, M. & Plotkin, S. Seasonal influenza vaccine efficacy and its determinants in children and non-elderly adults: a systematic review with meta-analyses of controlled trials. Vaccine 31 , 49–57 (2012).
Beyer, W. E. et al. Reply: Letter to the editor, Cochrane rearranged. Vaccine 33 , 13–14 (2015).
Centers for Disease Control and Prevention. ACIP votes down use of LAIV for 2016–2017 flu season. CDC https://www.cdc.gov/media/releases/2016/s0622-laiv-flu.html (2016).
Saito, N. et al. Negative impact of prior influenza vaccination on current influenza vaccination among people infected and not infected in prior season: a test-negative case-control study in Japan. Vaccine 35 , 687–693 (2017).
Paules, C. I., Sullivan, S. G., Subbarao, K. & Fauci, A. S. Chasing seasonal influenza - the need for a universal influenza vaccine. N. Engl. J. Med. 378 , 7–9 (2018).
Hata, A., Akashi-Ueda, R., Takamatsu, K. & Matsumura, T. Safety and efficacy of peramivir for influenza treatment. Drug. Des. Dev. Ther. 8 , 2017–2038 (2014).
McKimm-Breschkin, J. L. Influenza neuraminidase inhibitors: antiviral action and mechanisms of resistance. Influenza Other Respir. Viruses 7 (Suppl. 1), 25–36 (2013).
Okoli, G. N., Otete, H. E., Beck, C. R. & Nguyen-Van-Tam, J. S. Use of neuraminidase inhibitors for rapid containment of influenza: a systematic review and meta-analysis of individual and household transmission studies. PLOS ONE 9 , e113633 (2014).
Muthuri, S. G. et al. Effectiveness of neuraminidase inhibitors in reducing mortality in patients admitted to hospital with influenza A H1N1pdm09 virus infection: a meta-analysis of individual participant data. Lancet Respir. Med. 2 , 395–404 (2014).
Dobson, J., Whitley, R. J., Pocock, S. & Monto, A. S. Oseltamivir treatment for influenza in adults: a meta-analysis of randomised controlled trials. Lancet 385 , 1729–1737 (2015).
Venkatesan, S. et al. Impact of outpatient neuraminidase inhibitor treatment in patients infected with influenza A(H1N1)pdm09 at high risk of hospitalization: an individual participant data metaanalysis. Clin. Infect. Dis. 64 , 1328–1334 (2017).
Deyde, V. M. et al. Surveillance of resistance to adamantanes among influenza A(H3N2) and A(H1N1) viruses isolated worldwide. J. Infect. Dis. 196 , 249–257 (2007).
Gubareva, L. V. et al. Comprehensive assessment of 2009 pandemic influenza A (H1N1) virus drug susceptibility in vitro. Antivir. Ther 15 , 1151–1159 (2010).
Meijer, A. et al. Oseltamivir-resistant influenza virus A (H1N1), Europe, 2007–2008 season. Emerg. Infect. Dis. 15 , 552–560 (2009).
Bloom, J. D., Gong, L. I. & Baltimore, D. Permissive secondary mutations enable the evolution of influenza oseltamivir resistance. Science 328, 1272–1275.
Abed, Y., Pizzorno, A., Bouhy, X. & Boivin, G. Role of permissive neuraminidase mutations in influenza A/Brisbane/59/2007-like (H1N1) viruses. PLOS Pathog. 7 , e1002431 (2011).
Okomo-Adhiambo, M. et al. Oseltamivir-resistant influenza A(H1N1)pdm09 viruses, United States, 2013–2014. Emerg. Infect. Dis. 21 , 136–141 (2015).
Operario, D. J., Moser, M. J. & St George, K. Highly sensitive and quantitative detection of the H274Y oseltamivir resistance mutation in seasonal A/H1N1 influenza virus. J. Clin. Microbiol. 48 , 3517–3524 (2010).
Memoli, M. J., Hrabal, R. J., Hassantoufighi, A., Eichelberger, M. C. & Taubenberger, J. K. Rapid selection of oseltamivir- and peramivir-resistant pandemic H1N1 virus during therapy in 2 immunocompromised hosts. Clin. Infect. Dis. 50 , 1252–1255 (2010).
Whitley, R. J. et al. Global assessment of resistance to neuraminidase inhibitors, 2008-2011: the Influenza Resistance Information Study (IRIS). Clin. Infect. Dis. 56 , 1197–1205 (2013).
Butler, J. et al. Estimating the fitness advantage conferred by permissive neuraminidase mutations in recent oseltamivir-resistant A(H1N1)pdm09 influenza viruses. PLOS Pathog. 10 , e1004065 (2014).
Meijer, A. et al. Global update on the susceptibility of human influenza viruses to neuraminidase inhibitors, 2012–2013. Antiviral Res. 110 , 31–41 (2014).
Yamashita, M. Laninamivir and its prodrug, CS-8958: long-acting neuraminidase inhibitors for the treatment of influenza. Antivir. Chem. Chemother. 21 , 71–84 (2010).
Furuta, Y. et al. Favipiravir (T-705), a novel viral RNA polymerase inhibitor. Antiviral Res. 100 , 446–454 (2013).
Clark, M. P. et al. Discovery of a novel, first-in-class, orally bioavailable azaindole inhibitor (VX-787) of influenza PB2. J. Med. Chem. 57 , 6668–6678 (2014).
Portsmouth, S., Kawaguchi, K., Arai, M., Tsuchiya, K. & Uehara, T. Cap-dependent endonuclease inhibitor S-033188 for the treatment of influenza: results from a phase 3, randomized, double-blind, placebo- and active-controlled study in otherwise healthy adolescents and adults with seasonal influenza. Open Forum Infect. Dis. 4 , S744 (2017). This paper reports clinical trial results of baloxavir marboxil, a new influenza drug approved in Japan in 2018 .
Haffizulla, J. et al. Effect of nitazoxanide in adults and adolescents with acute uncomplicated influenza: a double-blind, randomised, placebo-controlled, phase 2b/3 trial. Lancet Infect. Dis. 14 , 609–618 (2014).
Beigel, J. H. et al. Immune plasma for the treatment of severe influenza: an open-label, multicentre, phase 2 randomised study. Lancet Respir. Med. 5 , 500–511 (2017).
Hung, I. F. N. et al. Hyperimmune IV immunoglobulin treatment: a multicenter double-blind randomized controlled trial for patients with severe 2009 influenza A(H1N1) infection. Chest 144 , 464–473 (2013).
Koszalka, P., Tilmanis, D. & Hurt, A. C. Influenza antivirals currently in late-phase clinical trial. Influenza Other Respir. Viruses 11 , 240–246 (2017).
Torner, N. et al. Effectiveness of non-pharmaceutical measures in preventing pediatric influenza: a case-control study. BMC Publ. Health 15 , 543 (2015).
Halloran, M. E. et al. Modeling targeted layered containment of an influenza pandemic in the United States. Proc. Natl Acad. Sci. USA 105 , 4639–4644 (2008).
Fiore, A. E. et al. Antiviral agents for the treatment and chemoprophylaxis of influenza — recommendations of the Advisory Committee on Immunization Practices (ACIP). MMWR Recomm. Rep. 60 , 1–24 (2011).
Uyeki, T. Antiviral treatment for patients hospitalized with 2009 pandemic influenza A (H1N1). N. Engl. J. Med. 361 , e110 (2009).
Stewart, R. J. et al. Influenza antiviral prescribing for outpatients with an acute respiratory illness and at high risk for influenza-associated complications during 5 influenza seasons-United States, 2011–2016. Clin. Infect. Dis. 66 , 1035–1041 (2018).
Oboho, I. K. et al. Oseltamivir use among children and adults hospitalized with community-acquired pneumonia. Open Forum Infect. Dis. 4 , ofw254 (2017).
Coleman, B. L. et al. Pre-and post-pandemic trends in antiviral use in hospitalized patients with laboratory-confirmed influenza: 2004/05-2013/14, Toronto, Canada. Antiviral Res. 140 , 158–163 (2017).
Hung, I. F. N. et al. Efficacy of clarithromycin-naproxen-oseltamivir combination in the treatment of patients hospitalized for influenza A(H3N2) infection: an open-label randomized, controlled, phase IIb/III trial. Chest 151 , 1069–1080 (2017).
Sims, L. D. Intervention strategies to reduce the risk of zoonotic infection with avian influenza viruses: scientific basis, challenges and knowledge gaps. Influenza Other Respir. Viruses 7 (Suppl. 2), 15–25 (2013).
Domenech, J. et al. Experiences with vaccination in countries endemically infected with highly pathogenic avian influenza: the Food and Agriculture Organization perspective. Rev. Sci. Tech. 28 , 293–305 (2009).
Ellis, T. M. et al. Use of avian influenza vaccination in Hong Kong. Dev. Biol. 124 , 133–143 (2006).
Leung, Y. H. et al. Avian influenza and ban on overnight poultry storage in live poultry markets, Hong Kong. Emerg. Infect. Dis. 18 , 1339–1341 (2012).
Lau, E. H. et al. Effect of interventions on influenza A (H9N2) isolation in Hong Kong’s live poultry markets, 1999–2005. Emerg. Infect. Dis. 13 , 1340–1347 (2007).
Bao, C. J. et al. Live-animal markets and influenza A (H7N9) virus infection. N. Engl. J. Med. 368 , 2337–2339 (2013).
Chambers, T. M., Dubovi, E. J. & Donis, R. O. in Textbook of Influenza 2nd edn (eds Webster, R. G., Monto, A. S., Braciale, T. J. & Lamb, R. A.) (Blackwell Science, Oxford, 2013).
Nichol, K. L., D’Heilly, S. J., Greenberg, M. E. & Ehlinger, E. Burden of influenza-like illness and effectiveness of influenza vaccination among working adults aged 50–64 years. Clin. Infect. Dis. 48 , 292–298 (2009).
Gozalo, P. L., Pop-Vicas, A., Feng, Z., Gravenstein, S. & Mor, V. Effect of influenza on functional decline. J. Am. Geriatr. Soc. 60 , 1260–1267 (2012).
Molinari, N. A. et al. The annual impact of seasonal influenza in the US: measuring disease burden and costs. Vaccine 25 , 5086–5096 (2007).
Rello, J. & Pop-Vicas, A. Clinical review: primary influenza viral pneumonia. Crit. Care 13 , 235 (2009).
Morens, D. M., Taubenberger, J. K. & Fauci, A. S. Predominant role of bacterial pneumonia as a cause of death in pandemic influenza: implications for pandemic influenza preparedness. J. Infect. Dis. 198 , 962–970 (2008).
Chien, Y. W., Klugman, K. P. & Morens, D. M. Bacterial pathogens and death during the 1918 influenza pandemic. N. Engl. J. Med. 361 , 2582–2583 (2009).
Morris, D. E., Cleary, D. W. & Clarke, S. C. Secondary bacterial infections associated with influenza pandemics. Front. Microbiol. 8 , 1041 (2017).
Taubenberger, J. K. & Morens, D. M. The pathology of influenza virus infections. Annu. Rev. Pathol. 3 , 499–522 (2008).
Agyeman, P., Duppenthaler, A., Heininger, U. & Aebi, C. Influenza-associated myositis in children. Infection 32 , 199–203 (2004).
MacDonald, K. L. et al. Toxic shock syndrome. A newly recognized complication of influenza and influenzalike illness. JAMA 257 , 1053–1058 (1987).
Steininger, C. et al. Acute encephalopathy associated with influenza A virus infection. Clin. Infect. Dis. 36 , 567–574 (2003).
Dourmashkin, R. R. What caused the 1918–1930 epidemic of encephalitis lethargica? J. R. Soc. Med. 90 , 515–520 (1997).
Zost, S. J. et al. Contemporary H3N2 influenza viruses have a glycosylation site that alters binding of antibodies elicited by egg-adapted vaccine strains. Proc. Natl Acad. Sci. USA 114 , 12578–12583 (2017).
Hayden, F. G. Experimental human influenza: observations from studies of influenza antivirals. Antivir. Ther. 17 , 133–141 (2012).
Erbelding, E. J. et al. A universal influenza vaccine: the strategic plan for the National Institute of Allergy and Infectious Diseases. J. Infect. Dis. https://doi.org/10.1093/infdis/jiy103 (2018).
Freidl, G. S. et al. Influenza at the animal-human interface: a review of the literature for virological evidence of human infection with swine or avian influenza viruses other than A(H5N1). Euro Surveill. 19 https://www.eurosurveillance.org/images/dynamic/EE/V19N18/art20793.pdf (2014).
Abdelwhab el, S. M., Veits, J. & Mettenleiter, T. C. Genetic changes that accompanied shifts of low pathogenic avian influenza viruses toward higher pathogenicity in poultry. Virulence 4 , 441–452 (2013).
World Health Organization. Cumulative number of confirmed human cases for avian influenza A(H5N1) reported to WHO, 2003–2017. WHO http://www.who.int/influenza/human_animal_interface/2017_12_07_tableH5N1.pdf (2017).
Pantin-Jackwood, M. J. et al. Role of poultry in the spread of novel H7N9 influenza virus in China. J. Virol. 88 , 5381–5390 (2014).
World Health Organization. Human infection with avian influenza A(H7N9) virus – China. WHO http://www.who.int/csr/don/26-october-2017-ah7n9-china/en/ (2017).
Imai, M. et al. A highly pathogenic avian H7N9 influenza virus isolated from A human is lethal in some ferrets infected via respiratory droplets. Cell Host Microbe 22 , 615–626 (2017).
Li, C. et al. Evolution of H9N2 influenza viruses from domestic poultry in Mainland China. Virology 340 , 70–83 (2005).
Lam, T. T. et al. The genesis and source of the H7N9 influenza viruses causing human infections in China. Nature 502 , 241–244 (2013).
Palese, P., Tumpey, T. M. & García-Sastre, A. What can we learn from reconstructing the extinct 1918 pandemic influenza virus? Immunity 24 , 121–124 (2006).
Matthey, S. et al. Rapid detection of respiratory viruses by shell vial culture and direct staining by using pooled and individual monoclonal antibodies. J. Clin. Microbiol. 30 , 540–544 (1992).
Loeffelholz, M. J. et al. Comparison of the FilmArray Respiratory Panel and Prodesse real-time PCR assays for detection of respiratory pathogens. J. Clin. Microbiol. 49 , 4083–4088 (2011).
Teo, J. et al. VereFlu: an integrated multiplex RT-PCR and microarray assay for rapid detection and identification of human influenza A and B viruses using lab-on-chip technology. Arch. Virol. 156 , 1371–1378 (2011).
Kim, D. K. & Poudel, B. Tools to detect influenza virus. Yonsei Med. J. 54 , 560–566 (2013).
Hurt, A. C., Alexander, R., Hibbert, J., Deed, N. & Barr, I. G. Performance of six influenza rapid tests in detecting human influenza in clinical specimens. J. Clin. Virol. 39 , 132–135 (2007).
Download references
Acknowledgements
All authors are part of the NIAID-funded Centers of Excellence for Influenza Research and Surveillance (CEIRS) network.
Reviewer information
Nature Reviews Disease Primers thanks R.W. Compans, F. Hayden, H. Kida, H.-D. Klenk, A. Osterhaus and the other anonymous reviewer(s) for their contribution to the peer review of this work.
Author information
Authors and affiliations.
Department of Microbiology, Icahn School of Medicine at Mount Sinai, New York, NY, USA
Florian Krammer, Peter Palese, Megan L. Shaw & Adolfo García-Sastre
Duke–NUS Medical School, Singapore, Singapore
Gavin J. D. Smith
Duke Global Health Institute, Duke University, Durham, NC, USA
Department of Viroscience, Erasmus MC, Rotterdam, Netherlands
Ron A. M. Fouchier
WHO Collaborating Centre for Infectious Disease Epidemiology and Control, School of Public Health, Li Ka Shing Faculty of Medicine, The University of Hong Kong, Hong Kong Special Administrative Region, Hong Kong, China
Malik Peiris
Center of Influenza Research, Li Ka Shing Faculty of Medicine, The University of Hong Kong, Hong Kong Special Administrative Region, Hong Kong, China
Department of Microbiology and Immunology, University of Melbourne at the Peter Doherty Institute for Infection and Immunity, Melbourne, Victoria, Australia
Katherine Kedzierska & Peter C. Doherty
Department of Immunology, St Jude Children’s Research Hospital, Memphis, TN, USA
Peter C. Doherty
Division of Infectious Diseases, Department of Medicine, Icahn School of Medicine at Mount Sinai, New York, NY, USA
Peter Palese & Adolfo García-Sastre
Division of Infectious Diseases, Department of Medicine, University of Rochester School of Medicine and Dentistry, Rochester, NY, USA
John Treanor
Department of Infectious Diseases, St Jude Children’s Research Hospital, Memphis, TN, USA
Robert G. Webster
Global Health and Emerging Pathogens Institute, Icahn School of Medicine at Mount Sinai, New York, NY, USA
Adolfo García-Sastre
You can also search for this author in PubMed Google Scholar
Contributions
Introduction (A.G.-S.); Epidemiology (G.J.D.S.); Mechanisms/pathophysiology (A.G.-S., R.A.M.F., M.P., F.K., K.K. and P.C.D.); Diagnosis, screening and prevention (P.P. and M.L.S.); Management (J.T. and R.G.W.); Quality of life (J.T.); Outlook (A.G.-S.); Overview of the Primer (A.G.-S.).
Corresponding author
Correspondence to Adolfo García-Sastre .
Ethics declarations
Competing interests.
F.K., P.P., A.G.-S. and R.G.W. are inventors of influenza vaccine technologies owned by the Icahn School of Medicine at Mount Sinai, New York, USA. All other authors declare no competing interests.
Additional information
Publisher’s note.
Springer Nature remains neutral with regard to jurisdictional claims in published maps and institutional affiliations.
Rights and permissions
Reprints and permissions
About this article
Cite this article.
Krammer, F., Smith, G.J.D., Fouchier, R.A.M. et al. Influenza. Nat Rev Dis Primers 4 , 3 (2018). https://doi.org/10.1038/s41572-018-0002-y
Download citation
Published : 28 June 2018
DOI : https://doi.org/10.1038/s41572-018-0002-y
Share this article
Anyone you share the following link with will be able to read this content:
Sorry, a shareable link is not currently available for this article.
Provided by the Springer Nature SharedIt content-sharing initiative
This article is cited by
Impaired influenza a virus replication by the host restriction factor samhd1 which inhibited by pa-mediated dephosphorylation of the host transcription factor irf3.
- Zhilei Zhao
- Hongxuan He
Virology Journal (2024)
The prediction of influenza-like illness using national influenza surveillance data and Baidu query data
BMC Public Health (2024)
The role of PB1-F2 in adaptation of high pathogenicity avian influenza virus H7N7 in chickens
- Luise Hohensee
- David Scheibner
- Ulrike Blohm
Veterinary Research (2024)
Vaccine design via antigen reorientation
- Joshua J. Carter
- Peter S. Kim
Nature Chemical Biology (2024)
Highly pathogenic avian influenza virus H5N1 clade 2.3.4.4b from Peru forms a monophyletic group with Chilean isolates in South America
- Gina R. Castro-Sanguinetti
- Rosa González-Veliz
- Juan A. More-Bayona
Scientific Reports (2024)
Quick links
- Explore articles by subject
- Guide to authors
- Editorial policies
Sign up for the Nature Briefing: Microbiology newsletter — what matters in microbiology research, free to your inbox weekly.

share this!
April 16, 2024
This article has been reviewed according to Science X's editorial process and policies . Editors have highlighted the following attributes while ensuring the content's credibility:
fact-checked
reputable news agency
Virologist offers perspective on avian influenza outbreak
by Deb Balzer, Mayo Clinic News Network
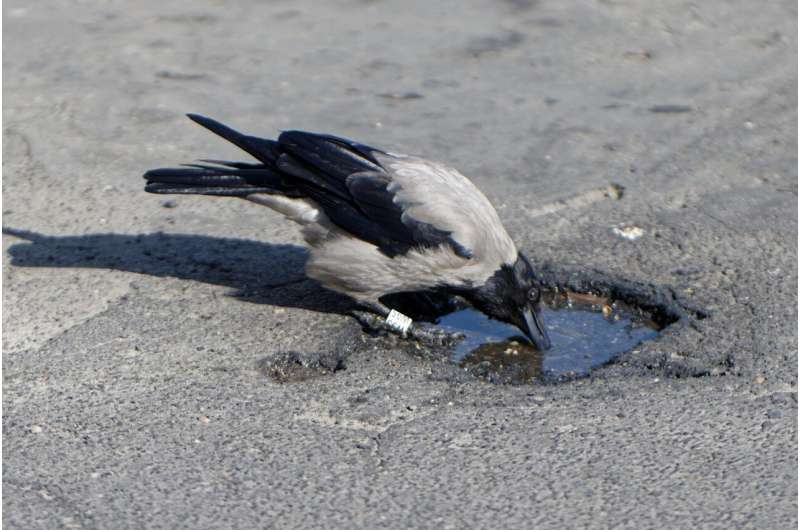
Health officials around the globe, including the Centers for Disease Control and Prevention and the European Center for Disease Control and Prevention, are monitoring the ongoing avian influenza outbreak. Also known as bird flu, the highly contagious viral disease typically spreads among birds, but can also infect livestock and, in rare cases, humans.
"Avian influenza is very common among migratory birds such as waterfowl," says Dr. Matthew Binnicker, director of the Clinical Virology Laboratory at Mayo Clinic. He adds that human infection is rare, occurring mainly through close contact with infected animals. Dr. Binnicker says that while the current risk to humans is low, it's crucial to take preventive measures and ensure diagnostic tools and treatments.
In this Q&A, Dr. Binnicker offers more context on the current influenza outbreak.
Q: What is the risk of bird flu transmitting to humans?
A: Currently, the CDC is characterizing the risk to humans of this avian influenza outbreak as low. So, if we're on a scale of 1 to 10, we're probably at a 1 or a 2. Now, the concern is that we're seeing not only millions of infections in commercial poultry and backyard bird flocks that humans frequently come into contact with, but we're also seeing higher rates of infection in mammals.
Q: How is avian influenza transmitted?
A: The birds will transmit avian influenza through their own respiratory mucosal secretions, much like we think about with other respiratory viruses in humans, or through contaminated feces. So a bird can become infected through exposure to contaminated soil or feces.
For humans, the transmission so far has been very rare.
Q: What are the symptoms of infection?
Those individuals were infected by coming into very close contact with animals who had avian influenza. These individuals may have come in contact with a respiratory secretion from an infected bird or mammal, or a contaminated surface. It is possible for humans to inhale the virus, or to become infected through self-inoculation if they touch a contaminated surface and then introduce the virus into their eyes, nose or mouth.
A: In the wild, migratory birds often do not show symptoms of avian influenza infection. Wild birds are a reservoir for avian influenza viruses. In commercial poultry and backyard bird flocks, that's where we see more severe disease . Since 2022, there have been over 60 million commercial poultry or backyard bird flocks killed or culled because of avian influenza infection.
In humans, avian influenza can present in a similar way to normal human influenza infection. As is the case with other respiratory viral infections, avian influenza in humans may cause a spectrum of illness, from mild disease to a severe lower respiratory tract infection. In the recent case in Texas, the patient only experienced conjunctivitis, which is redness and inflammation in the eyes.
Q: Can bird flu be prevented?
A: The key preventive measure is to avoid contact with a sick or dead animal, especially birds. If you own a backyard bird flock or work in a commercial poultry facility, and there are birds that are sick or have died, do not come in contact with them.
And if it's impossible to avoid contact, it's important to take necessary precautions, including wearing eye protection, an N95 mask and gloves. Those protective measures are going to help prevent an individual from coming into contact with avian influenza virus, either through inhalation of infected respiratory secretions or by self-inoculating the virus from a contaminated surface into the eyes, nose or mouth.
Q: What should the public keep in mind?
A: Currently, the overall risk of widespread infection in humans is low. We know that this virus is transmitted efficiently among wild birds , and since 2022, it has resulted in millions of infections in commercial poultry and backyard bird flocks. We've also started to see infection in many types of mammals, but so far, infection in humans is rare.
We need to approach this with a sense of preparedness. Now is the time to put tools in place in the event we see sustained, high rates of transmission in humans. These tools include testing, antivirals and vaccines, which can be rapidly deployed and hopefully prevent avian influenza from becoming a worldwide problem.
2024 Mayo Clinic News Network. Distributed by Tribune Content Agency, LLC.
Explore further
Feedback to editors

Study reveals how humanity could unite to address global challenges
2 hours ago

CO₂ worsens wildfires by helping plants grow, model experiments show
4 hours ago

Surf clams off the coast of Virginia reappear and rebound
5 hours ago

Yellowstone Lake ice cover unchanged despite warming climate

The history of the young cold traps of the asteroid Ceres
6 hours ago

Researchers shine light on rapid changes in Arctic and boreal ecosystems

New benzofuran synthesis method enables complex molecule creation

Human odorant receptor for characteristic petrol note of Riesling wines identified

Uranium-immobilizing bacteria in clay rock: Exploring how microorganisms can influence the behavior of radioactive waste

Research team identifies culprit behind canned wine's rotten egg smell
Relevant physicsforums posts, can four legged animals drink from beneath their feet.
Apr 15, 2024
Mold in Plastic Water Bottles? What does it eat?
Apr 14, 2024
Dolphins don't breathe through their esophagus
Is this egg-laying or something else.
Apr 13, 2024
Color Recognition: What we see vs animals with a larger color range
Apr 12, 2024
How to Implement Beamforming in Ultrasound Diffraction Tomography
Apr 10, 2024
More from Biology and Medical
Related Stories

Bird flu: What is it, how does it spread and how can we protect ourselves from it?
Mar 25, 2024

Protecting poultry from bird flu
Nov 30, 2023

Clinic monitoring rising avian influenza cases, preparing for potential human-to-human outbreak
Mar 14, 2023

Severe human infection with a novel avian-origin influenza A(H7N4) virus
Sep 4, 2018

Uptick in avian flu cases poses little threat to humans
May 19, 2022

What is avian flu, the disease afflicting viral TikTok emu Emmanuel?
Oct 17, 2022
Recommended for you

Research team shows island bats are valuable allies for farmers

Older male blue tits out-compete young males when it comes to extra-marital breeding
7 hours ago

Exploring the interactions between baby marmosets and their caregivers
11 hours ago

Climate-change-driven cold snaps threaten marine life
12 hours ago

Twisted pollen tubes induce infertility in plants with multiple sets of chromosomes
Let us know if there is a problem with our content.
Use this form if you have come across a typo, inaccuracy or would like to send an edit request for the content on this page. For general inquiries, please use our contact form . For general feedback, use the public comments section below (please adhere to guidelines ).
Please select the most appropriate category to facilitate processing of your request
Thank you for taking time to provide your feedback to the editors.
Your feedback is important to us. However, we do not guarantee individual replies due to the high volume of messages.
E-mail the story
Your email address is used only to let the recipient know who sent the email. Neither your address nor the recipient's address will be used for any other purpose. The information you enter will appear in your e-mail message and is not retained by Phys.org in any form.
Newsletter sign up
Get weekly and/or daily updates delivered to your inbox. You can unsubscribe at any time and we'll never share your details to third parties.
More information Privacy policy
Donate and enjoy an ad-free experience
We keep our content available to everyone. Consider supporting Science X's mission by getting a premium account.
E-mail newsletter
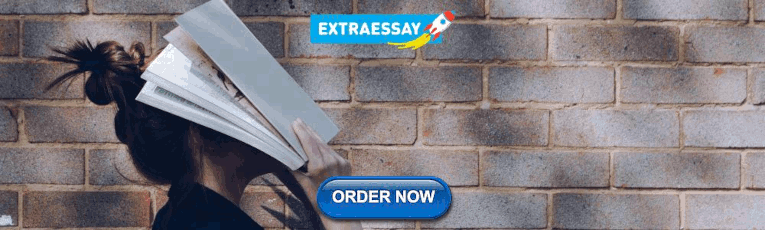
IMAGES
VIDEO
COMMENTS
Abstract. Although antiviral drugs and vaccines have reduced the economic and healthcare burdens of influenza, influenza epidemics continue to take a toll. Over the past decade, research on ...
Annual seasonal influenza epidemics of variable severity caused by influenza A and B virus infections result in substantial disease burden worldwide. Seasonal influenza virus circulation declined markedly in 2020-21 after SARS-CoV-2 emerged but increased in 2021-22. Most people with influenza have abrupt onset of respiratory symptoms and myalgia with or without fever and recover within 1 ...
Influenza is a cause of significant morbidity, mortality, economic and social disruption. Annual seasonal influenza epidemics result in 290,000-650,000 deaths worldwide, while influenza pandemics have resulted in many more - the A(H1N1) pandemic of 1918-1919 caused 20-50 million deaths. ... Research and innovation. The COVID-19 pandemic ...
January 31, 2024 - A recent CDC study suggests that vaccination with non-egg-based flu vaccines might improve the antibody response to circulating flu viruses over that of traditional egg-based vaccines, which are the most common flu vaccines worldwide. The study looked at people's immune response to various flu vaccines to determine which ...
Influenza vaccine [in-activated vaccines], split virion, egg-based vaccines (AQ) Afluria-quadrivalent: Seqirus-Pty. Ltd. USA ... Nevertheless, there is a need for further research to address the limitations of current influenza vaccines, such as their limited efficacy, lengthy production processes, and lack of broad cross-protection. ...
The further adaptation of these viruses to replication in, and transmission among, humans may lead to the eventual development of a pandemic virus. Four influenza pandemics have emerged in the past century, causing the Spanish flu (1918, H1N1), the Asian flu (1957, H2N2), the Hong Kong flu (1968, H3N2), and the swine-origin flu pandemic of 2009 ...
Further research is warranted to elucidate the precise mechanisms driving this association and to explore the long-term impact of influenza vaccination on cardiovascular outcomes.
Please use one of the following formats to cite this article in your essay, paper or report: APA. Bose, Priyom. (2024, April 15). Study shows potential for universal flu vaccine with broad ...
Future research of high-quality randomized controlled trials is recommended to further assess the efficacy of the influenza vaccine in COVID-19 patients. The regular updating of the influenza ...
1 Introduction. Seasonal influenza carries a significant burden, causing up to 650,000 deaths annually across the globe as well as billions of dollars' worth of direct and indirect costs to individuals and society [1, 2].Rapid viral evolution lies behind the toll of influenza as new strains capable of evading the human immune response continually arise due to antigenic drift, in which ...
Season cohort sizes ranged from 887,260 to 3,628,168 individuals. Of all patient characteristics evaluated, the cumulative number of CDC-defined high-risk influenza conditions that an individual had was most predictive of increased probability of having an IRME overall and across age groups, with adults of any age with ORs for influenza hospitalization ranging from 1.8 (95%CI: 1.7 to 2.0) for ...
The lack of effective antivirals has pushed influenza research towards vaccine development, using the immune system's robust antiviral response against the virus, as opposed to therapeutics. Still, there are reasons to continue the search for truly effective antivirals. ... This work may further improve seasonal vaccines, creating a virtuous ...
Influenza vaccination was associated with illness attenuation among those hospitalised with influenza, although results differed by vaccine target group. These findings might suggest that attenuation of disease severity might be specific to certain target groups, seasons, or settings. ... However, further research is needed to investigate these ...
Therefore, further understanding of and improving influenza immunization for older adults remain a priority in translational aging research. The seven original research articles and one review in this Topical Collection cover a number of fundamental and frontier research topics relevant to influenza immunization research in human aging.
2 Emerging influenza threat and bio-risk reduction. Influenza infection, caused by influenza viruses, is common, seasonal, and global (Kumar et al., 2018; Asha and Kumar, 2019).The disease is transmitted by inhalation of aerosols containing virions via the respiratory mucosa (Iwasaki and Pillai, 2014; Asha et al., 2021).More than one hundred years ago in 1918, a pandemic emerged during World ...
1 Federal State Budget Institution "National Research Centre for Epidemiology and Microbiology Named after Honorary Academician N. F. Gamaleya" of the Ministry of Health of the Russian Federation, Moscow, Russia; 2 Department of Virology, Lomonosov Moscow State University, Moscow, Russia; 3 Department of Medical Genetics, I. M. Sechenov First Moscow State Medical University, Moscow, Russia
Influenza Information for Researchers. Colorized structure of a prototype for a universal flu vaccine. NIAID is conducting and supporting research to find new and improved ways to diagnose, treat and prevent influenza infection. This includes working toward a universal flu vaccine that could provide long-lasting protection against multiple ...
Influenza causes approximately 15,000-70,000 deaths annually in Europe, mainly in older adults [], and complications such as exacerbations of underlying pulmonary disease or bacterial co-infections further increase the burden of disease [5, 7].Vaccination against influenza is recommended in many countries for everybody, but is particularly relevant for older adults and other risk groups.
Pandemic influenza A viruses arise as a result of antigenic shift; that is, the expression of novel HA and/or NA proteins. 3 Such a dramatic change results in viruses that are distinct from ...
Further research on transmission modes and alternative interventions to reduce influenza transmission would be valuable in improving pandemic preparedness. Finally, although our review focused on nonpharmaceutical measures to be taken during influenza pandemics, the findings could also apply to severe seasonal influenza epidemics.
Further research should explore the optimization and customization of search terms for different regions and languages to improve the accuracy of influenza prediction models. Keywords: early warning; epidemic intelligence; infectious disease; influenza-like illness; surveillance.
NIAID has a longstanding commitment to conducting and supporting the basic research necessary to understand how influenza strains emerge, evolve, infect and cause disease (called pathogenesis) in animals and humans. Results from this research are used to inform the design of new and improved influenza vaccines, diagnostics and antiviral drugs ...
Influenza is an acute respiratory illness, caused by influenza A, B, and C viruses, that occurs in local outbreaks or seasonal epidemics. Clinical illness follows a short incubation period and presentation ranges from asymptomatic to fulminant, depending on the characteristics of both the virus and the individual host. Influenza A viruses can also cause sporadic infections or spread worldwide ...
The trial was established to assess seasonal influenza vaccine efficacy in school-aged children, 6-17 years old, initially from 119 households, including 71 households in which a child received ...
For influenza type A, at least 16 highly variable hemagglutinins (H1 to H16) and 9 distinct NAs (N1 to N9) have been recognized so far. With the aid of these different antigens, the influenza type A virus is further subdivided into subtypes on the basis of variable combination patterns of their own specific H or N proteins (e.g., H1N1 or H3N2).
April 5, 2024 —On March 29, 2024, CDC reported this year's first U.S. human infection with an influenza (flu) virus that normally spreads in pigs and not people. The infection with an influenza A(H1N2) variant (v) virus occurred in a child living near a pig farm in Pennsylvania who had direct contact with pigs prior to illness onset. The person was hospitalized and has since recovered from ...
However, further characterization provided evidence that it was genetically, antigenically and biologically distinct from human ICV . In August 2016, the International Committee on Taxonomy of Viruses (ICTV) classified this novel virus as a new genus (Deltainfluenzavirus, species: Influenza D virus) of the family Orthomyxoviridae.
Scientific Reports (2024) Influenza is an infectious respiratory disease that, in humans, is caused by influenza A and influenza B viruses. Typically characterized by annual seasonal epidemics ...
Nanoparticles show great promise as a platform for developing vaccines for the prevention of infectious disease. We have been investigating a method whereby nanocapsules can be formulated from protein, such that the final capsules contain only the cross-linked protein itself. Such nanocapsules are made using a silica templating system and can be customised in terms of size and porosity.
Virologist offers perspective on avian influenza outbreak. Health officials around the globe, including the Centers for Disease Control and Prevention and the European Center for Disease Control ...