- Architecture and Design
- Asian and Pacific Studies
- Business and Economics
- Classical and Ancient Near Eastern Studies
- Computer Sciences
- Cultural Studies
- Engineering
- General Interest
- Geosciences
- Industrial Chemistry
- Islamic and Middle Eastern Studies
- Jewish Studies
- Library and Information Science, Book Studies
- Life Sciences
- Linguistics and Semiotics
- Literary Studies
- Materials Sciences
- Mathematics
- Social Sciences
- Sports and Recreation
- Theology and Religion
- Publish your article
- The role of authors
- Promoting your article
- Abstracting & indexing
- Publishing Ethics
- Why publish with De Gruyter
- How to publish with De Gruyter
- Our book series
- Our subject areas
- Your digital product at De Gruyter
- Contribute to our reference works
- Product information
- Tools & resources
- Product Information
- Promotional Materials
- Orders and Inquiries
- FAQ for Library Suppliers and Book Sellers
- Repository Policy
- Free access policy
- Open Access agreements
- Database portals
- For Authors
- Customer service
- People + Culture
- Journal Management
- How to join us
- Working at De Gruyter
- Mission & Vision
- De Gruyter Foundation
- De Gruyter Ebound
- Our Responsibility
- Partner publishers

Your purchase has been completed. Your documents are now available to view.
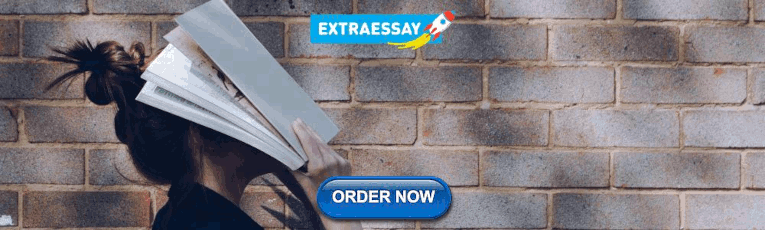
Green synthesis of nanoparticles: current prospectus
Tejaswi Thunugunta, DST INSPIRE fellow at the Indian Institute of Horticultural Research, Bangalore, India, is working on nanobiotechnology. Her major area of interest is to study the effect of nanoparticles on plants. She has published two papers on nanoparticle synthesis and their antimicrobial activity in international journals, during her postgraduation project work.
Anand C. Reddy is a research scholar at the Indian Institute of Horticultural Research and a postgraduate of Nottingham Trent University, UK. He has a keen interest in molecular biology and nanotechnology. He is currently working on gene delivery mechanisms in plants, mediated by nanoparticles. He is also working on molecular marker-assisted selection (MAS) in plants.
Lakshmana Reddy D.C. has a MS in Agriculture with a specialization in Plant Biotechnology, and a PhD in Agricultural Biotechnology. He has 8 years’ experience as a scientist in the Agricultural Research Service, Indian Council of Agricultural Research (ICAR). He has published in more than 25 national and international journals. Currently, he is working on molecular marker development, utilization in various horticulture crops for trait improvement, and nanobiotechnology applications for the improvement of horticultural crop production and productivity.
In the past few years, nanoparticles have been applied in various fields of science and technology, ranging from material science to biotechnology. Thus, the synthesis of nanoparticles can be considered as a dynamic area in research and application of nanoparticles. The different methods of nanoparticle synthesis include physical, chemical, and biological methods. Of these methods, the biological synthesis is to be comparatively widely used due to its advantages of being low cost, nontoxic and environmental friendly. Bio-applications of nanoparticles have pawed way for green synthesis of nanoparticles. In this review, we have provided brief information on various biological agents used for the synthesis of nanoparticles.
About the authors

Acknowledgments
This work is supported by the Department of Science and Technology, Government of India.
Conflict of interests: There is no competing interest on this review.
[1] Tadeusz M, Waldemar K, Marek F, Jussi K, Pavel Z. Human Factors of a Global Society, A System of Systems Perspective, Technology and Engineering , CRC Press, London, June 2, 2014, pp. 108. Search in Google Scholar
[2] Fendler JH, Ed., Nanoparticles and Nanostructured Films, Preparation, Characterization and Applications , John Wiley & Son: New York, NY, USA, 1998. Search in Google Scholar
[3] Parashar UK, Saxena PS, Srivastava A. Bioinspired synthesis of silver nanoparticles. Dig. J. Nanomater. Biostruct . 2009, 41, 159–166. Search in Google Scholar
[4] Schmidt-Ott A. New approaches to in situ characterization of ultrafine agglomerates. J. Aerosol. Sci . 1988, 195, 553–557, 559–563. Search in Google Scholar
[5] Håkansson G, Hultman L, Sundgren JE. Microstructures of TiN films grown by various physical vapour deposition techniques. Surf. Coat. Technol . 1991, 481, 51–67. Search in Google Scholar
[6] Sun Y, Fuge GM, Ashfold MNR, Growth of aligned ZnO nanorod arrays by catalyst-free pulsed laser deposition methods. Chem. Phys. Lett . 2004, 3961–3963, 21–26. Search in Google Scholar
[7] Zhu J, Palchik O, Chen S, Gedanken A. Microwave assisted preparation of CdSe, PbSe, and Cu 2- x Se nanoparticles. J. Phys. Chem. B . 2000, 10431, 7344–7347. Search in Google Scholar
[8] Chen Y, Fitz Gerald J, Williams JS, Bulcock S. Synthesis of boron nitride nanotubes at low temperatures using reactive ball milling. Chem. Phys. Lett . 1999, 2993–2994, 260–264. Search in Google Scholar
[9] Bikiaris DN, Papageorgiou GZ, Pavlidou E, Vouroutzis N, Palatzoglou P, Karayannidis GP. Preparation by melt mixing and characterization of isotactic polypropylene/SiO 2 nanocomposites containing untreated and surface-treated nanoparticles. J. Appl. Polym. Sci. 2006, 1004, 2684–2696. Search in Google Scholar
[10] Mafuné F, Kohno J, Takeda Y, Kondow T. Structure and stability of silver nanoparticles in aqueous solution produced by laser ablation. J. Phys. Chem. B . 2000, 10435, 8333–8337. Search in Google Scholar
[11] Stepanov AL, Hole DE, Townsend PD. Formation of silver nanoparticles in soda-lime silicate glass by ion implantation near room temperature. J. Non Cryst. Solids 1999, 2601–2602, 65–74. Search in Google Scholar
[12] Ayyub P, Chandra R, Taneja P, Sharma AK, Pinto R. Synthesis of nanocrystalline material by sputtering and laser ablation at low temperatures. Appl. Phys. A. 2001, 731, 67–73. Search in Google Scholar
[13] Journet C, Maser WK, Bernier P, Loiseau A, Lamy de la Chapelle M, Lefrant S, Deniard P, Lee R, Fischer JE, Large-scale production of single-walled carbon nanotubes by the electric-arc technique. Nature 1997, 388, 756–758. Search in Google Scholar
[14] Park J, Kwon SG, Jun SW, Kim BH, Hyeon T. Large-scale synthesis of ultra-small-sized silver nanoparticles. Chem. Phys. Chem. 2012, 13, 2540–2543. Search in Google Scholar
[15] Chen D-H, He X-R. Synthesis of nickel ferrite nanoparticles by sol-gel method. Mater. Res. Bull . 2001, 36, 1369–1377. Search in Google Scholar
[16] Kim YI, Kim D, Lee CS. Synthesis and characterization of CoFe 2 O 4 magnetic nanoparticles prepared by temperature-controlled coprecipitation method. Physica B: Condens. Matter 2003, 337, 42–51. Search in Google Scholar
[17] Cassell AM, Raymakers JA, Kong J, Dai H. Large scale CVD synthesis of single-walled carbon nanotubes. J. Phys. Chem. B . 1999, 103, 6484–6492. Search in Google Scholar
[18] Li J-G, Ikeda M, Ye R, Moriyoshi Y, Ishigaki T. Control of particle size and phase formation of TiO 2 nanoparticles synthesized in RF induction plasma. J. Phys. D: Appl. Phys. 2007, 40, 2348. Search in Google Scholar
[19] Li B, Xie Y, Huang J, Qian Y. Synthesis by a solvothermal route and characterization of CuInSe 2 nanowhiskers and nanoparticles. Adv. Mater . 1999, 11, 1456–1459. Search in Google Scholar
[20] Singh AK, Viswanath V, Janu VC. Synthesis, effect of capping agents, structural, optical and photoluminescence properties of ZnO nanoparticles. India J. Lumin. 2009, 1298, 874–878. Search in Google Scholar
[21] Mohanpuri P, Rana NK, Yadav SK. Biosynthesis of nanoparticles, technological concepts and future applications. J. Nanopart. Res. 2008, 10, 507–517. Search in Google Scholar
[22] Jang E, Ryu BH, Shim HW, Ju H, Kim DW, Kim TD. Adsorption of microbial esterases on Bacillus subtilis -templated cobalt oxide nanoparticles. Int. J. Biol. Macromol . 2014, 65, 188–192. Search in Google Scholar
[23] Ahmad A, Mukherjee P, Mandal D, Senapati S, Khan MI, Kumar R, Sastry M. Enzyme mediated extracellular synthesis of CdS nanoparticles by the fungus, Fusarium oxysporum . J. Am. Chem. Soc. 2002, 124, 12108–12109. Search in Google Scholar
[24] Shukla R, Nune SK, Chanda N, Katti K, Mekapothula S, Kulkarni RR, Welshons WV, Kannan R, Katti KV. Soybeans as a phytochemical reservoir for the production and stabilization of biocompatible gold nanoparticles. Small 2008, 4, 1425–1436. Search in Google Scholar
[25] Lee J, Park EY, Lee J. Non-toxic nanoparticles from phytochemicals, preparation and biomedical application. Bioproc. Biosyst. Eng . 2014, 376, 983–989. Search in Google Scholar
[26] Raveendran P, Fu J, Wallen SL. Completely green synthesis and stabilization of metal nanoparticles. J. Am. Chem. Soc. 2003, 12546, 13940–13941. Search in Google Scholar
[27] Inbakandan D, Venkatesan R, Khan SA. Biosynthesis of gold nanoparticles utilizing marine sponge Acanthella elongata . Colloid. Surface B 2010, 812, 634–639. Search in Google Scholar
[28] Nellorea J, Paulineb PC, Amarnath K. Biogenic synthesis by S phearanthus amaranthoids towards the efficient production of the biocompatible gold nanoparticles. Dig. J. Nanomater. Bios. 2012, 7, 123–133. Search in Google Scholar
[29] Tejaswi T, Venkateshwara Rao K, Shilpa C. Sliver nanoparticles synthesis and stabilization by different species of ocimum and characterization for its antimicrobial activity. Int. J. Curr. Eng. Tech. 2013, 32, 501–506. Search in Google Scholar
[30] Klaus T, Joerger R, Olsson E, Granqvist CG. Silver-based crystalline nanoparticles, microbially fabricated. Proc. Natl. Acad. Sci. USA 1999, 9624, 13611–13614. Search in Google Scholar
[31] Mohseniazar M, Barin M, Zarredar H, Alizadeh S, Shanehbandi D. Potential of microalgae and lactobacilli in biosynthesis of silver nanoparticles. BioImpacts 2011, 1, 149–152. Search in Google Scholar
[32] Gericke M, Pinches A. Biological synthesis of metal nanoparticles. Hydrometallurgy 2006, 83, 132–140. Search in Google Scholar
[33] Husseiny MI, EI-Aziz MA, Badr Y, Mahmoud MA. Biosynthesis of gold nanoparticles using Pseudomonas aeruginosa. Spectrochim. Acta Part A 2007, 673–674, 1003–1006. Search in Google Scholar
[34] Fu JZ, Liu YY, Gu PY, Shang DL, Lin ZY, Yao BX, Weng SZ. Spectroscopic characterization on the biosorption and bioreduction of AgI by Lactobacillus sp. A09. Acta Physico. Chim. Sin. 2000, 169, 770–782. Search in Google Scholar
[35] Joerger R, Klaus T, Granqvist CG. Biologically produced Ag-C composite materials for optically functional thin film coatings. Adv. Mat. 2000, 12, 407–409. Search in Google Scholar
[36] Mukherjee P, Ahmad A, Mandal D. Bioreduction of AuCl4- ions by the fungus, Verticillium sp. and surface trapping of the gold nanoparticles formed. Angew. Chem. Int. Ed. Engl. 2001, 40, 3585–3588. Search in Google Scholar
[37] Shankar SS, Rai A, Ahmad A, Sastry M. Rapid synthesis of Au, Ag and bimetallic Au core- Ag shell nanoparticles using neem Azadirachtaindica leaf broth. J. Colloid Interf. Sci . 2004, 275, 496–502. Search in Google Scholar
[38] Ganesh Babu MM, Gunasekaran P. Production and structural characterization of crystalline silver nanoparticles from Bacillus cereus isolate. Colloid. Surface B 2009, 74, 191–195. Search in Google Scholar
[39] Jain D, Kachhwaha S, Jain R, Srivastava G, Kothari SL. Novel microbial route to synthesize silver nanoparticles using spore crystal mixture of Bacillus thuringiensis . Indian J. Exp. Biol. 2010, 48, 1152–1156. Search in Google Scholar
[40] Gurunathan S, Kalishwaralal K, Vaidyanathan R, Venkataraman D, Pandian SR, Muniyandi J, Hariharan N, Eom SH. Biosynthesis, purification and characterization of silver nanoparticles using Escherichia coli. Colloid. Surface B 2009, 74, 328–335. Search in Google Scholar
[41] Sweeney RY, Mao C, Gao X, Burt JL, Belcher AM, Georgiou G, Iverson BL. Bacterial biosynthesis of cadmium sulfide nanocrystals. Chem. Biol. 2004, 11, 1553–1559. Search in Google Scholar
[42] Sintubin L, De Windt W, Dick J, Mast J, van der Ha D, Verstraete W, Boon N. Lactic acid bacteria as reducing and capping agent for the fast and efficient production of silver nanoparticles. Appl. Microbiol. Biotechnol. 2009, 84, 741–749. Search in Google Scholar
[43] Zhang H, Li Q, Lu Y, Sun D, Lin X, Deng X, He N, Zheng S. Biosorption and bioreduction of diamine silver complex by Corynebacterium. J. Chem. Technol. Biotechnol. 2005, 80, 285–290. Search in Google Scholar
[44] Nanda A, Saravanan M. Biosynthesis of silver nanoparticles from Staphylococcus aureus and its antimicrobial activity against MRSA and MRSE. Nanomedicine. 2009, 5, 452–456. Search in Google Scholar
[45] Juibari MM, Abbasalizadeh S, Jouzani GS, Noruzi M. Intensified biosynthesis of silver nanoparticles using a native extremophilic Ureibacillus thermosphaericus strain. Mater. Lett. 2011, 65, 1014–1017. Search in Google Scholar
[46] Fu JK, Zhang WD, Liu YY, Lin ZY, Yao BX, Weng SZ, Zeng JL. Characterization of adsorption and reduction of noble metal ions by bacteria. Chem. J. Chinese U. 1999, 20, 1452–1454. Search in Google Scholar
[47] Parikh RP, Singh S, Prasad BLV, Patole MS, Sastry M, Shouche YS. Extracellular synthesis of crystalline silver nanoparticles and molecular evidence of silver resistance from Morganella sp towards understanding biochemical synthesis mechanism. Chembiochem. 2008, 9, 1415–1422. Search in Google Scholar
[48] Nair B, Pradeep T. Coalescence of nanoclusters and formation of submicron crystallites assisted by Lactobacillus strains. Cryst. Growth Des. 2002, 2, 293–298. Search in Google Scholar
[49] Lengke M, Fleet M, Southam G. Biosynthesis of silver nanoparticles by filamentous cyanobacteria from a silver I nitrate complex. Langmuir 2006, 10, 1021–1030. Search in Google Scholar
[50] Cunningham DP, Lundie LL. Precipitation of cadmium by Clostridium thermoaceticum. Appl. Environ. Microbiol. 1993, 9, 7–14. Search in Google Scholar
[51] Bharde A, Wani A, Shouche Y, Pattayil A, Bhagavatula L, Sastry M. Bacterial aerobic synthesis of nanocrystalline magnetite. JACS 2005, 127, 9326–9327. Search in Google Scholar
[52] Konishi Y, Ohno K, Saitoh N, Nomura T, Nagamine S. Microbial synthesis of gold nanoparticles by metal reducing bacterium. Trans. Mater. Res. Soc. Jpn. 2004, 29, 2341–2343. Search in Google Scholar
[53] Shiying H, Zhirui G, Zhanga Y, Zhanga S, Wanga J, Ning G. Biosynthesis of gold nanoparticles using the bacteria Rhodopseudomonas capsulata. Mater. Lett. 2007, 61, 3984–3987. Search in Google Scholar
[54] Liangwei D, Hong J, Xiaohua L, Erkang W. Biosynthesis of gold nanoparticles assisted by Escherichia coli DH5α and its application on direct electrochemistry of hemoglobin. Electrochem. Commun. 2007, 9, 1165–1170. Search in Google Scholar
[55] Ahmad A, Senapati S, Khan MI, Kumar R, Sastry M. Extracellular biosynthesis of monodisperse gold nanoparticles by a novel extremophilic actinomycete, Thermomonospora sp. Langmuir 2003, 19, 3550–3553. Search in Google Scholar
[56] Ahmad A, Senapati S, Khan MI, Ramani R, Srinivas V, Sastry M. Intracellular synthesis of gold nanoparticles by a novel alkalotolerant actinomycete, Rhodococcus species. Nanotechnology 2003, 14, 824–828. Search in Google Scholar
[57] Shahverdi AR, Fakhimi A, Shahverdi HR, Minaian S. Synthesis and effect of silver nanoparticles on the antibacterial activity of different antibiotics against Staphylococcus aureus and Escherichia coli. Nanomedicine 2007, 3, 168–171. Search in Google Scholar
[58] Husseiney MI, Abd El-Aziz M, Badr Y, Mahmoud MA. Biosynthesis of gold nanoparticles using Pseudomonas aeruginosa. Spectrochim. Acta A. 2007, 67, 1003–1006. Search in Google Scholar
[59] Marshall M, Beliaev A, Dohnalkova A, David W, Shi L, Wang Z, Boyanov MI, Lai B, Kemner KM, McLean JS, Reed SB, Culley DE, Bailey VL, Simonson CJ, Saffarini DA, Romine MF, Zachara JM, Fredrickson JK. c-Type cytochrome-dependent formation of UIV nanoparticles by Shewanella oneidensis. PLoS Biol. 2007, 8, 1324–1333. Search in Google Scholar
[60] Vijayakumar PS, Prasad BLV. Intracellular biogenic silver nanoparticles for the generation of carbon supported antiviral and sustained bactericidal agents. Langmuir 2009, 25, 11741–11747. Search in Google Scholar
[61] Kalimuthu K, Suresh Babu R, Venkataraman D, Bilal M, Gurunathan S. Biosynthesis of silver nanocrystals by Bacillus licheniformis. Colloid. Surface B 2008, 65, 150–153. Search in Google Scholar
[62] Shahverdi AR, Minaeian S, Shahverdi HR, Jamalifar H, Nohi AA. Rapid synthesis of silver nanoparticles using culture supernatants of Enterobacteriaceae: a novel biological process. Biochem. 2007, 42, 919–923. Search in Google Scholar
[63] Varshney R, Bhadauria S, Gaur MS, Pasricha R. Copper nanoparticles synthesis from electroplating industry effluent. Nano. Biomed. Eng. 2011, 3, 115–119. Search in Google Scholar
[64] Balagurunathan R, Radhakrishnan M, Babu Rajendranand R, Velmurugan D. Biosynthesis of gold nanoparticles by actinomycetes Streptomyces viridogens strain HM10. Indian J. Biochem. Biophys. 2011, 48, 331–335. Search in Google Scholar
[65] Sharma N, Pinnaka AK, Raje M, Fnu A, Bhattacharyya MS, Choudhury AR. Exploitation of marine bacteria for production of gold nanoparticles. Microb. Cell Fact. 2012, 11, 86. Search in Google Scholar
[66] Yeary LW, Moon JW, Love LJ, Thompson JR, Rawn CJ, Phelps TJ. Magnetic properties of biosynthesized magnetite nanoparticles. IEEE T. Magn. 2005, 41, 4384–4389. Search in Google Scholar
[67] Yeary LW, Moon JW, Rawn CJ, Love LJ, Rondinone AJ, Thompson JR, Chakoumakos BC, Phelps TJ. Magnetic properties of bio-synthesized zinc ferrite nanoparticles. J. Magn. Magn. Mater . 2011, 323, 3043–3048. Search in Google Scholar
[68] Sundaram PA, Augustine R, Kannan M. Extracellular biosynthesis of iron oxide nanoparticles by Bacillus subtilis strains isolated from rhizosphere soil. Biotechnol. Bioprocess Eng. 2012, 4, 835–840. Search in Google Scholar
[69] Malarkodi C, Rajeshkumar S, Paulkumar K, Vanaja M, Jobitha GDG, Annadurai G. Bactericidal activity of bio mediated silver nanoparticles synthesized by Serratia nematodiphila. Drug. Invention Today 2013, 5, 119–125. Search in Google Scholar
[70] Lee JH, Roh Y, Hur HG. Microbial production and characterization of superparamagnetic magnetite nanoparticles by Shewanella sp. HN-41. J. Microbiol. Biotechn. 2008, 18, 1572–1577. Search in Google Scholar
[71] Du L, Jiang H, Liu X, Wang E. Biosynthesis of gold nanoparticles assisted by Escherichia coli DH5α and its application on direct electrochemistry of hemoglobin. Electrochem. Commun. 2007, 9, 1165–1170. Search in Google Scholar
[72] He S, Guo Z, Zhang Y, Zhang S, Wang J, Gu N. Biosynthesis of gold nanoparticles using the bacteria Rhodopseudomonas capsulate. Mater. Lett. 2007, 61, 3984–3987. Search in Google Scholar
[73] Kalishwaralal K, Deepak V, Ram Kumar Pandian S, Kottaisamy M, BarathmaniKanth S, Kartikeyan B, Gurunathan S. Biosynthesis of silver and gold nanoparticles using Brevibacterium casei. Colloid. Surface B 2010, 77, 257–262. Search in Google Scholar
[74] Sinha A, Khare SK. Mercury bioaccumulation and simultaneous nanoparticle synthesis by Enterobacter sp. Cells. Bioresource Technol. 2011, 102, 4281–4284. Search in Google Scholar
[75] Bao H, Lu Z, Cui X, Qiao Y, Guo J, Anderson JM, Li CM. Extracellular microbial synthesis of biocompatible CdTe quantum dots. Acta Biomaterialia. 2010, 6, 3534–3541. Search in Google Scholar
[76] Kashefi K, Lovley DR. Reduction of FeIII, MnIV, and toxic metals at 100°C by Pyrobaculum islandicum. Appl. Environ. Microbiol . 2000, 66, 1050–1056. Search in Google Scholar
[77] Lloyd JR, Yong P, Macaskie LE. Enzymatic recovery of elemental palladium by using sulfate-reducing bacteria. Appl. Environ. Microbiol . 1998, 64, 4607–4609. Search in Google Scholar
[78] Amemiya Y, Arakaki A, Staniland SS, Tanaka T, Matsunaga T. Controlled formation of magnetite crystal by partial oxidation of ferrous hydroxide in the presence of recombinant magnetotactic bacterial protein Mms6. Biomaterials . 2007, 28, 5381–5389. Search in Google Scholar
[79] Li W, Yu L, Zhou P, Zhu M. A Magnetospirillum strain WM-1 from a freshwater sediment with intracellular magnetosomes. World J. Microbiol. Biotechn. 2007, 23, 1489–1492. Search in Google Scholar
[80] Jha AK, Prasad K. Ferroelectric BaTiO3 nanoparticles, biosynthesis and characterization. Colloid. Surface B 2010, 75, 330–334. Search in Google Scholar
[81] Jha AK, Prasad K, Kulkarni AR. Synthesis of TiO2 nanoparticles using microorganisms. Colloid. Surface B 2009, 71, 226–229. Search in Google Scholar
[82] Zhu K, Pan H, Li J, Yu-Zhang K, Zhang SD, Zhang WY, Zhou K, Yue H, Pan Y, Xiao T, Wu LF. Isolation and characterization of a marine magnetotactic spirillum axenic culture QH-2 from an intertidal zone of the China Sea. Res. Microbiol . 2010, 161, 276–283. Search in Google Scholar
[83] Arakaki A, Shibusawa M, Hosokawa M, Matsunaga T. Preparation of genomic DNA from a single species of uncultured magnetotactic bacterium by multiple-displacement amplification. Appl. Environ. Microbiol . 2010, 76, 1480–1485. Search in Google Scholar
[84] Bai HJ, Zhang ZM, Guo Y, Yang GE. Biosynthesis of cadmium sulfide nanoparticles by photosynthetic bacteria Rhodopseudomonas palustris. Colloid. Surface B 2009, 70, 142–146. Search in Google Scholar
[85] Prasad K, Jha AK. Biosynthesis of CdS nanoparticles, an improved green and rapid procedure. J. Colloid Interf. Sci . 2010, 342, 68–72. Search in Google Scholar
[86] Bai HJ, Zhang ZM, Gong J. Biological synthesis of semiconductor zinc sulfide nanoparticles by immobilized Rhodobacter sphaeroides . Biotechnol. Lett . 2006, 28, 1135–1139. Search in Google Scholar
[87] Labrenz M, Druschel GK, Thomsen-Ebert T, Gilbert B, Welch SA, Kemner KM, Logan GA, Summons RE, De Stasio G, Bond PL, Lai B, Kelly SD, Banfield JF. Formation of sphalerite ZnS deposits in natural biofilms of sulfate-reducing bacteria. Science 2000, 290, 1744–1747. Search in Google Scholar
[88] Bai HJ, Zhang ZM. Microbial synthesis of semiconductor lead sulfide nanoparticles using immobilized Rhodobacter sphaeroides. Mater. Lett. 2009, 63, 764–766. Search in Google Scholar
[89] Watson JHP, Ellwood DC, Soper AK, Charnock J. Nanosized strongly-magnetic bacterially-produced iron sulfide materials. J. Magn. Magn. Mater . 1999, 203, 69–72. Search in Google Scholar
[90] Pandian SRK, Deepak V, Kalishwaralal K, Muniyandi J, Rameshkumar N, Gurunathan S. Synthesis of PHB nanoparticles from optimized medium utilizing dairy industrial waste using Brevibacterium casei SRKP2, a green chemistry approach. Colloid. Surface B 2009, 74, 266–273. Search in Google Scholar
[91] Sastry M, Ahmad A, Khan MI, Kumar R. Biosynthesis of metal nanoparticles using fungi and actinomycete. Curr. Sci. 2003, 852, 162–170. Search in Google Scholar
[92] Gade AK, Bonde PP, Ingle AP, Marcato P, Duran N, Rai MK. Exploitation of Aspergillus niger for synthesis of silver nanoparticles. J. Biobased Mater. Bio . 2008, 2, 1–5. Search in Google Scholar
[93] Binupriya AR, Sathishkumar M, Yun SI. Myco-crystallization of silver ions to nanosized particles by live and dead cell filtrates of Aspergillus oryzae var. viridis and its bactericidal activity toward Staphylococcus aureus KCCM 12256. Ind Eng Chem Res . 2010, 49, 852–858. Search in Google Scholar
[94] Duran N, Marcato PD, De Souza GIH, Alves OL, Esposito E. Antibacterial effect of silver nanoparticles produced by fungal process on textile fabrics and their effluent treatment. J. Biomed. Nanotechnol . 2007, 3, 203–208. Search in Google Scholar
[95] Ingle A, Rai M, Gade A, Bawaskar M. Fusarium solani, A novel biological agent for the extracellular synthesis of silver nanoparticles. J. Nanopart. Res . 2009, 11, 2079–2085. Search in Google Scholar
[96] Nithya R, Ragunathan R. Synthesis of silver nanoparticles using Pleurotus sajor caju and its antimicrobial study. Dig. J. Nanomater. Bios. 2009, 4, 623–629. Search in Google Scholar
[97] Thakkar KN, Mhatre SS, Parikh RY. Biological synthesis of metallic nanoparticles. Nanomedicine . 2010, 6, 257–262. Search in Google Scholar
[98] FesharakiI PJ, NazariI P, ShakibaieI M, RezaieII S, Banoee M, AbdollahiM, ShahverdiAR. Biosynthesis of selenium nanoparticles using Klebsiella pneumoniae and their recovery by a simple sterilization process. Braz. J. Microbiol . 2010, 41, 461–466. Search in Google Scholar
[99] Chen JC, Lin ZH, Ma XX. Evidence of the production of silver nanoparticles via pretreatment of Phoma sp.3.2883 with silver nitrate. Lett. Appl. Microbiol . 2003, 37, 105–108. Search in Google Scholar
[100] Ahmad A, Mukherjee P, Senapati S, Mandal D, Khan MI, Kumar R, Sastry M. Extracellular biosynthesis of silver nanoparticles using the fungus Fusarium oxysporum . Colloid. Surface B 2003, 28, 313–318. Search in Google Scholar
[101] Mukherjee P, Ahmad A, Mandal D, Senapati S, Sainkar SR, Khan MI, Parishcha R, Ajaykumar PV, Alam M, Kumar R, Sastry M. Fungus mediated synthesis of silver nanoparticles and their immobilization in the mycelial matrix, a novel biological approach to nanoparticle synthesis. Nano Lett . 2001, 1, 515–519. Search in Google Scholar
[102] Bhainsa KC, D’Souza SF. Extracellular biosynthesis of silver nanoparticle using the fungus Aspergillus fumigates. Colloid. Surface B 2006, 47, 160–164. Search in Google Scholar
[103] Mukherjee P, Roy M, Mandal B, Dey G, Mukherjee P, Ghatak J, Tyagi AK, Kale SP. Green synthesis of highly stabilized nanocrystalline silver particles by a non-pathogenic and agriculturally important fungus T. asperellum . Nanotechnology 2008, 19, 75103–75110. Search in Google Scholar
[104] Vigneshwaran N, Kathe AA, Varadarajan PV, Nachane RP, Balasubramanya RH. Biomimetics of Ag nanoparticles by white rot fungus, Phaenerochaete chrysosporium . Colloid. Surface B 2006, 53, 55–59. Search in Google Scholar
[105] Bharde A, Rautaray D, Bansal V, Ahmad A, Sarkar I, Yusuf SM, Sanyal M, Sastry M. Extracellular biosynthesis of magnetite using fungi. Small 2006, 21, 135–141. Search in Google Scholar
[106] Vigneshwaran N, Ashtaputre NM, Varadarajan PV, Nachane RP, Paralikar KM, Balasubramanya RH. Biological synthesis of silver nanoparticles using the fungus Aspergillus flavus . Mater. Lett. 2007, 61, 1413–1418. Search in Google Scholar
[107] Ingle A, Gade A, Pierrat S, Sonnichsen C, Rai M. Mycosynthesis of silver nanoparticles using the fungus Fusarium acuminatum and its activity against some human pathogenic bacteria. Current Nanoscience . 2008, 4, 141–144. Search in Google Scholar
[108] Basavaraja SS, Balaji SD, Lagashetty AK, Rajasab AH, Venkataraman A. Extracellular biosynthesis of silver nanoparticles using the fungus Fusarium semitectum . Mater. Res. Bull . 2008, 43, 1164–1170. Search in Google Scholar
[109] Li G, He D, Qian Y, Guan B, Gao S, Cui Y, Yokoyama K, Wang L. Fungus-mediated green synthesis of silver nanoparticles using Aspergil lusterreus . Int. J. Mol. Sci. 2012, 13, 466–476. Search in Google Scholar
[110] Bansal V, Rautaray D, Ahmad A, Sastry M. Biosynthesis of zirconia nanoparticles using the fungus Fusarium oxysporum. J. Mater. Chem . 2004, 14, 3303–3305. Search in Google Scholar
[111] Tarafdar JC, Raliya R. Rapid, low-cost, and ecofriendly approach for iron nanoparticle synthesis using Aspergillus oryzae TFR9. J. Nanopart. 2013, 4 pages. Search in Google Scholar
[112] Agnihotri M, Joshi S, Kumar AR, Zinjarde S, Kulkarni S. Biosynthesis of gold nanoparticles by the tropical marine yeast Yarrowia lipolytica NCIM 3589. Mater. Lett. 2009, 63, 1231–1234. Search in Google Scholar
[113] Castro-Longoria E, Vilchis-Nestor AR, Avalos Borja M. Biosynthesis of silver, gold and bimetallic nanoparticles using the filamentous fungus Neurospora crassa . Colloid. Surface B 2011, 83, 42–48. Search in Google Scholar
[114] Bansal V, Rautaray D, Bharde A, Ahire K, Sanyal A, Ahmad A, Sastry M. Fungus-mediated biosynthesis of silica and titania particles. J. Mater. Chem . 2005, 15, 2583–2589. Search in Google Scholar
[115] Bansal V, Poddar P, Ahmad A, Sastry M. Room temperature biosynthesis of ferroelectric barium titanate nanoparticles. J. Am. Chem. Soc . 2006, 128, 11958–11963. Search in Google Scholar
[116] Sanghi R, Verma P. A facile green extracellular biosynthesis of CdS nanoparticles by immobilized fungus. Chem. Eng. J . 2009, 155, 886–891. Search in Google Scholar
[117] Kowshik M, Deshmuke N, Vogal W, Urban J, Kulkarni SK, Paknikar KM. Microbial synthesis of semiconductor CdS nanoparticles, their characterization, and their use in the fabrication of an ideal diode. Biotechnol Bioeng. 2002, 78, 583–588. Search in Google Scholar
[118] Dameron CT, Reese RN, Mehra RK, Kortan AR, Carroll PJ, Steigerwald ML, Brus LE, Winge DR. Biosynthesis of cadmium sulphide quantum semiconductor crystallites. Nature 1989, 338, 596–597. Search in Google Scholar
[119] Sanyal A, Rautaray D, Bansal V, Ahmad A, Sastry M. Heavy-metal remediation by a fungus as a means of production of lead and cadmium carbonate crystals. Langmuir 2005, 21, 7220–7224. Search in Google Scholar
[120] Rautaray D, Sanyal A, Adyanthaya SD, Ahmad A, Sastry M. Biological synthesis of strontium carbonate crystals using the fungus Fusarium oxysporum . Langmuir 2004, 20, 6827–6833. Search in Google Scholar
[121] Kumar SA, Ansary AA, Abroad A, Khan MI. Extracellular biosynthesis of CdSe quantum dots by the fungus, Fusarium oxysporum . J. Biomed. Nanotechnol . 2007, 32, 190–194. Search in Google Scholar
[122] Sanghi R, Verma P. Biomimetic synthesis and characterization of protein capped silver nanoparticles. Bioresource Technol. 2009, 100, 501–504. Search in Google Scholar
[123] Balaji DS, Basavaraja S, Deshpande R, Mahesh DB, Prabhakar BK, Venkataraman A. Extracellular biosynthesis of functionalized silver nanoparticles by strains of Cladosporium cladosporioides fungus. Colloid. Surface B 2009, 68, 88–92. Search in Google Scholar
[124] Kowshik M, Ashtaputre S, Kharrazi S, Vogel W, Urban J, Kulkarni SK, Paknikar KM. Extracellular synthesis of silver nanoparticles by a silver-tolerant yeast strain MKY3. Nanotechnology 2003, 14, 95–100. Search in Google Scholar
[125] Mourato A, Gadanho M, Lino AR, Tenreiro R. Biosynthesis of crystalline silver and gold nanoparticles by extremophilic yeasts. Bioinorg Chem Appl . 2011, 546074. Search in Google Scholar
[126] Seshadri S, Saranya K, Kowshik M. Green synthesis of lead sulfide nanoparticles by the lead resistant marine yeast, Rhodosporidium diobovatum . Biotechnol. Progr. 2011, 27, 1464–1469. Search in Google Scholar
[127] Zhou W, He W, Zhong S, Wang Y, ZhaoH, Li Z, Yan S. Biosynthesis and magnetic properties of mesoporous Fe3O4 composites. J. Magn. Magn. Mater . 2009, 321, 1025–1028. Search in Google Scholar
[128] Zhou W, He W, Zhang X, Yan S, Sun X, Tian X, Han X. Biosynthesis of iron phosphate nanopowders. Powder Technol. 2009, 194, 106–108. Search in Google Scholar
[129] Jha AK, Prasad K. A green low-cost biosynthesis of Sb2O3 nanoparticles. Biochem. Eng. J . 2009, 43, 303–306. Search in Google Scholar
[130] Yan S, He W, Sun C, Zhang X, Zhao H, Li Z, Zhou W, Tian X, Sun X, Han X. The biomimetic synthesis of zinc phosphate nanoparticles. Dyes Pigments 2009, 80, 254–258. Search in Google Scholar
[131] Thirumalairaj VK, Vijayan MP, Durairaj G, Shanmugaasokan L, Yesudas R, Gunasekara S. Potential antibacterial activity of crude extracts and silver nanoparticles synthesized from Sargassum wightii. Int. Curr. Pharm. J. 2014, 3, 322–325. Search in Google Scholar
[132] Luna C, Ilyn M, Vega V, Prida VM, González J, Mendoza-Reséndez R. Size distribution and frustrated antiferromagnetic coupling effects on the magnetic behavior of ultrafine akaganéite (β-FeOOH) nanoparticles. J. Phys. Chem. C , 2014, 118, 21128–21139. Search in Google Scholar
[133] Dhas TS, Kumar VG, Karthick V, Angel KJ, Govindaraju K. Facile synthesis of silver chloride nanoparticles using marine alga and its antibacterial efficacy. Spectrochim. Acta A 2014, 120, 416–420. Search in Google Scholar
[134] Singaravelu G, Arockiamary JS, Ganesh Kumar V, Govindaraju K. A novel extracellular synthesis of monodisperse gold nanoparticles using marine alga, Sargassum wightii Greville. Colloid. Surface B 2007, 57, 97–101. Search in Google Scholar
[135] Lengke MF, Fleet ME, Southam G. Morphology of gold nanoparticles synthesized by filamentous cyanobacteria from goldI-thiosulfate and goldIII-chloride complexes. Langmuir 2006, 22, 2780–2787. Search in Google Scholar
[136] Lengke MF, Ravel B, Fleet ME, Wanger G, Gordon RA, Southam G. Mechanisms of gold bioaccumulation by filamentous cyanobacteria from goldIII-chloride complex. Environ. Sci. Technol . 2006, 40, 6304–6309. Search in Google Scholar
[137] Konishi Y, Tsukiyama T, Tachimi T, Saitoh N, Nomura T, Nagamine S. Microbial deposition of gold nanoparticles by the metal-reducing bacterium Shewanella algae. Electrochim. Acta 2007, 53, 186–192. Search in Google Scholar
[138] Konishi Y, Ohno K, Saitoh N, Nomura T, Nagamine S, Hishida H, Takahashi Y, Uruga T. Bioreductive deposition of platinum nanoparticles on the bacterium Shewanella algae. J. Biotechnol . 2007, 128, 648–653. Search in Google Scholar
[139] Lee JH, Han J, Choi H, Hur HG. Effects of temperature and dissolved oxygen on SeIV removal and SeO precipitation by Shewanella sp. HN-41. Chemosphere 2007, 68, 1898–1905. Search in Google Scholar
[140] Perez-Gonzalez T, Jimenez-Lopez C, Neal AL, Rull-Perez F, Rodriguez-Navarro A, Fernandez-Vivas A, Iañez-Pareja E. Magnetite biomineralization induced by Shewanella oneidensis. Geochim. Cosmochim. Ac . 2010, 74, 967–979. Search in Google Scholar
[141] Bose S, Hochella MF, Gorby YA, Kennedy DW, McCready DE, Madden AS, Lower BH. Bioreduction of hematite nanoparticles by the dissimilatory iron reducing bacterium Shewanella oneidensis MR-1. Geochim. Cosmochim. Ac . 2009, 73, 962–976. Search in Google Scholar
[142] Lefèvre CT, Abreu F, Schmidt ML, Lins U, Frankel RB, Hedlund BP, Bazylinski DA Moderately thermophilic magnetotactic bacteria from hot springs in Nevada. Appl. Environ. Microbiol . 2010, 76, 3740–3743. Search in Google Scholar
[143] Huang J, Li Q, Sun D, Lu Y, Su Y, Yang X, Wang H, Wang Y, Shao W, He N, Hong J, Chen C. Biosynthesis of silver and gold nanoparticles by novel sundried Cinnamomum camphora leaf. Nanotechnology 2007, 18, 11–15. Search in Google Scholar
[144] Leela A, Vivekanandan M. Tapping the unexploited plant resources for the synthesis of silver nanoparticles. Afr. J. Biotechnol. 2007, 7, 3162–3165. Search in Google Scholar
[145] Manimekalai G, Selvakumar S, Gopikrishnan V, Radhakrishnan M, Balagurunathan R. Antibacterial effect of medicinal plants Leucas aspera Linn and Ocimum basilicum Linn. Int. J. Adv. Pharmaceut. Res . 2011, 26, 276–280. Search in Google Scholar
[146] Ahmad A, Senapati S, Khan MI, Kumar R, Ramani R, Srinivas V, Sastry M. Intracellular synthesis of gold nanoparticles by a novel alkalotolerantactinomycete, Rhodococcus species. Nanotechnology 2003, 14, 824–828. Search in Google Scholar
[147] Torresday JLG, Parsons JG, Gomez E, Videa JP, Troiani HE, Santiago P, Yacaman MJ. Formation and growth of Au nanoparticles inside live alfa alfa plants. Nanoletters 2002, 24, 397–401. Search in Google Scholar
[148] Chandran SP, Chaudhary M, Pasricha R, Ahmad A, Sastry M. Synthesis of gold nanotriangles and silver nanoparticles using Aloe vera plant extract. Biotechnol. Prog. 2006, 22, 577–583. Search in Google Scholar
[149] Vijayaraghavan K, Kamala Nalini SP, Udaya P, Rakash N, Madhankumar D. Biomimetic synthesis of silver nanoparticles by aqueous extract of Syzygium aromaticum. Mater. Lett. 2012, 75, 33–35. Search in Google Scholar
[150] Christensen L, Vivekanandhan S, Misra M, Mohanty A. Biosynthesis of silver nanoparticles using Murraya koenigii curry leaf. An investigation on the effect of broth concentration in reduction mechanism and particle size. Adv. Mat. Lett. 2011, 2, 429–434. Search in Google Scholar
[151] Patil CD, Patil SV, Borase HP, Salunke BK, Salunkhe RB. Larvicidal activity of silver nanoparticles synthesised using Plumeria rubra plant latex against Aedes aegypti and Anopheles stephensi. Parasitol. Res. 2012, 110, 1815–1822. Search in Google Scholar
[152] Pala R, Pathipati UR, Bojja S. Qualitative assessment of silver and gold nanoparticle synthesis in various plants. A photobiological approach. J. Nanopart. Res. 2010, 12, 1711–1721. Search in Google Scholar
[153] Shankar SS, Rai A, Ahmad A, Sastry M. Biosynthesis of silver and gold nanoparticles from extracts of different parts of the Geranium plant. Applications Nanotechnology 2004, 1, 69–77. Search in Google Scholar
[154] Daizy P. Green synthesis of gold and silver nanoparticles using Hibiscus rosa sinensis. Physica E . 2010, 42, 1417–1424. Search in Google Scholar
[155] Shankar S, Rai A, Ankamwar B, Singh A, Ahmad A, Sastry M. Biological synthesis of triangular gold nanoprisms. Nat. Mater. 2004, 3, 482–488. Search in Google Scholar
[156] Armendariz V, Herrera I, Peralta-Videa JR, Jose-Yacaman M, Troiani H, Santiago P, Gardea-Torresdey JL. Size controlled gold nanoparticles formation by Avena sativa biomass, use of plants in nanobiotechnology. J. Nanopart. Res. 2004, 6, 377–82. Search in Google Scholar
[157] Torresdey JL, Gomez E, Peralta-Videa JR, Parsons JG, Troiani H, Jose-Yacaman M. Alfalfa sprouts, a natural source for the synthesis of silver nanoparticles. Langmuir 2003, 19, 1357–1361. Search in Google Scholar
[158] Parida UK, Bindhani BK, Nayak P. Green synthesis and characterization of gold nanoparticles using onion Allium cepa extract. World J. NanoSci. Eng. 2011, 1, 93–98. Search in Google Scholar
[159] Von White II G, Kerscher P, Brown RM, Morella JD, McAllister W, Dean D, Kitchens CL. Green synthesis of robust, biocompatible silver nanoparticles using garlic extract. J. Nanomater . 2012, 12, 730746-58. Search in Google Scholar
[160] Kalidasan M, Yogamoorthi A. Biosynthesis of silver nanoparticles using Achyranthus aspera and its characterization. Int. J. Nanomater. Biostructures 2014, 41, 5–11. Search in Google Scholar
[161] Sheny DS, Mathew J, Philip D. Phytosynthesis of Au, Ag and Au-Ag bimetallic nanoparticles using aqueous extract and dried leaf of Anacardium occidentale. Spectrochim Acta A Mol Biomol Spectrosc . Int. Res. J. Biological Sci . 2013, 26, 66–76. Search in Google Scholar
[162] Sulochana S, Krishnamoorthy P, Sivaranjani K. Synthesis of silver nanoparticles using leaf extract of Andrographis paniculata. J. Pharmacol. Toxicol. 2012, 7, 251–258. Search in Google Scholar
[163] Kora AJ, Arunachala J. Green fabrication of silver nanoparticles by gum Tragacanth Astragalus gummifer . A dual functional reductant and stabilizer. J. Nanomater . 2012, 8 pages, Article ID 869765, doi, 10.1155/2012/869765. Search in Google Scholar
[164] Thirumurugan A, Jiflin GJ, Rajagomathi G, Tomy NA, Ramachandran S, Jaiganesh R. Biotechnological synthesis of gold nanoparticles of Azadirachta indica leaf extract. Int. J. Biol. Tech . 2010, 1, 75–77. Search in Google Scholar
[165] Boruah SK, Boruah PK, Sarma P, Medhi C, Medhi OK. Green synthesis of gold nanoparticles using Camellia sinensis and kinetics of the reaction. Adv. Mat. Lett . 2012, 3, 481–486. Search in Google Scholar
[166] Jain D, Daima HK, Kachhwaha S, Kothari SL. Synthesis of plant-mediated silver nanoparticles using papaya fruit extract and evaluation of their antimicrobial activities. Dig. J. Nanomater. Bios . 2009, 4, 557–563. Search in Google Scholar
[167] Palaniselvam K, Velanganni AAJ, Govindan SN, Karthi. Leaf assisted bioreduction of silver ions using leaves of Centella asiatica L. and its bioactivity. E-J. Life Sci . 2012, 1, 46–49. Search in Google Scholar
[168] Dwivedi AD, Gopal K. Plant- mediated biosynthesis of silver and gold nanoparticles. J. Biomed. Nanotechnol . 2011, 7, 163–164. Search in Google Scholar
[169] Vanaja M, Annadurai G. Coleus aromaticus leaf extract mediated synthesis of silver nanoparticles and its bactericidal activity. Appl. Nanosci. 2013, 3, 217–223. Search in Google Scholar
[170] Sathishkumar M, Sneha K, Kwak IS, Mao J, Tripathy SJ, Yun YS. Phyto-crystallization of palladium through reduction process using Cinnamom zeylanicum bark extract. J. Hazard Mater . 2009, 171, 400–404. Search in Google Scholar
[171] Xin Y, Qingbiao L, Huixuan W, Jiale H, Liqin L, Wenta W, Daohua S, Yuanbo S, James O, Luwei H, Yuanpeng W, Ning H, Lishan J. Green synthesis of palladium nanoparticles using broth of Cinnamomum camphora leaf. J. Nanopart. Res. 2010, 12, 1589–1598. Search in Google Scholar
[172] Satyavani K, Ramanathan T, Gurudeeban S. Plant mediated synthesis of biomedical silver nanoparticles by using leaf extract of Citrullus colocynthis. J. Nanosci. Nanotechno. 2011, 1, 95–101. Search in Google Scholar
[173] Kesharwani J, Yoon KY, Hwang J, Rai M. Phytofabrication of silver nanoparticles by leaf extract of Datura metel , hypothetical mechanism involved in synthesis. J. Bionanosci . 2009, 3, 39–44. Search in Google Scholar
[174] Ahmad N, Sharma S, Singh VN, Shamsi SF, Fatma A, Mehta BR. Biosynthesis of silver nanoparticles from Desmodium triflorum . A novel approach towards weed utilization. Biotechnol. Res. Int . 2011, Article ID 454090, 8 pages. doi, 10.4061/2011/454090. Search in Google Scholar
[175] Song JY, Kwon EY, Kim BS. Biological synthesis of platinum nanoparticles using Diopyros kaki leaf extract. Bioprocess. Biosyst. Eng. 2010, 33, 159–164. Search in Google Scholar
[176] Ghosh S, Patil S, Ahire M, Kitture R, Kale S, Pardesi K, Cameotra SS, Bellare J, Dhavale DD, Jabgunde A, Chopade AB. Synthesis of silver nanoparticles using Dioscorea bulbifera tuber extract and evaluation of its synergistic potential in combination with antimicrobial agents. Int. J. Nanomed. 2012, 7, 483–496. Search in Google Scholar
[177] Maheswari RU, Prabha AL, Nandagopalan V, Anburaja V. Green synthesis of silver nanoparticles by using rhizome extract of Dioscorea oppositifolia L. and their anti microbial activity against human pathogens. IOSR J. Pharma. Biol. Sci. 2012, 1, 38–42. Search in Google Scholar
[178] Gnanajobitha G, Annadurai G, Kannan C. Green synthesis of silver nanoparticles using Elettaria cardamomom and assessment of its antimicrobial activity. Int. J. Pharma. Sci. Res. 2012, 3, 323–330. Search in Google Scholar
[179] Jia L, Zhang Q, Li Q, Song H. The biosynthesis of palladium nanoparticles by antioxidants in Gardenia jasminoides Ellis, long lifetime nanocatalysts for pnitrotoluene hydrogenation. Nanotech. 20. 2012, doi, 10.1088/0957-4484/20/38/385601. Search in Google Scholar
[180] Dinesh S, Karthikeyan S, Arumugam P. Biosynthesis of silver nanoparticles from Glycyrrhiza glabra root extract. Arch. Appl. Sci. Res. 2012, 4, 178–187. Search in Google Scholar
[181] Bindhu MR, Umadevi M. Synthesis of monodispersed silver nanoparticles using Hibiscus cannabinus leaf extract and its antimicrobial activity. Spectrochim Acta A Mol. Biomol. Spectrosc. 2012, 101, 184–190. Search in Google Scholar
[182] Sable N, Gaikwad S, Bonde S, Gade A, Rai MM. Phytofabrication of silver nanoparticles by using aquatic plant Hydrilla verticilata. Nus. Biosci. 2012, 4, 45–49. Search in Google Scholar
[183] Hudlikar M, Joglekar S, Dhaygude M, Kodam K. Latex-mediated synthesis of ZnS nanoparticles, green synthesis approach. J. Nano. Res. 2012, 14, 1–6. Search in Google Scholar
[184] Fazaludeena MF, Manickamb C, Ashankytyc MAI, Ahmedd MQ, Beg QZ. Synthesis and characterizations of gold nanoparticles by Justicia gendarussa Burm F leaf extract. J. Microbiol. Biotech. Res. 2012, 2, 23–34. Search in Google Scholar
[185] Sivakumar P, Nethradevi C, Renganathan S. Synthesis of silver nanoparticles using Lantana camara fruit extract and its effect on pathogens. Asian J. Pharm. Clin. Res. 2012, 5, 97–101. Search in Google Scholar
[186] Im AR, Han L, Kim ER, Kim J, Kim YS, Park Y. Enhanced antibacterial activities of Leonuri herba extracts containing silver nanoparticles. Phytother. Res. 2012, 26, 1249–1255. Search in Google Scholar
[187] Aromal SA, Vidhu VK, Philip D. Green synthesis of well-dispersed gold nanoparticles using Macrotyloma uniflorum. Spectrochim Acta A Mol. Biomol. Spectrosc. 2012, 85, 99–104. Search in Google Scholar
[188] Ali DM, Thajuddin N, Jeganathan K, Gunasekaran M. Plant extract mediated synthesis of silver and gold nanoparticles and its antibacterial activity against clinically isolated pathogens. Colloid. Surface B 2011, 85, 360–365. Search in Google Scholar
[189] Vankar PS, Bajpai D. Preparation of gold nanoparticles from Mirabilis jalapa flowers. Indian J. Biochem. Biophys. 2010, 47, 157–160. Search in Google Scholar
[190] Mary EJ, Inbathamizh L. Green synthesis and characterization of nano silver using leaf extract of Morinda pubescens. Asian J. Pharm. Clin. Res. 2012, 5, 159–162. Search in Google Scholar
[191] Ahmad N, Sharma S, Alam MK, Singh VN, Shamsi SF, Mehta BR, Fatma A. Rapid synthesis of silver nanoparticles using dried medicinal plant of basil. Colloid. Surface B 2010, 81, 81–86. Search in Google Scholar
[192] Ashok kumar D. Rapid and green synthesis of silver nanoparticles using the leaf extracts of Parthenium hysterophorus . A novel biological approach. Int. Res. J. Pharma. 2012, 3, 169–173. Search in Google Scholar
[193] Sundaravadivelan C, Nalini M. Biolarvicidal effect of phyto-synthesized silver nanoparticles using Pedilanthus tithymaloides L. Poit stem extract against the dengue vector Aedes aegypti L. diptera. Culicidae. Asian Pac. J. Trop Biomed. 2011, 1–8. Search in Google Scholar
[194] Mallikarjuna K, Dillip GR, Narashima G, Sushma NJ, Raju BDP. Phyto-fabrication and characterization of silver nanoparticles from Piper betel broth. Res. J. Nanosci. Nanotech. 2012, 2, 17–23. Search in Google Scholar
[195] Garg S. Rapid biogenic synthesis of silver nanoparticles using black pepper Piper nigrum corn extract. Int. J. Innov. Biol. Chem. Sci. 2012, 3, 5–10. Search in Google Scholar
[196] Nabikhan A, Kandasamy K, Raj A, Alikunhi MN. Synthesis of antimicrobial silver nanoparticles by callus and leaf extracts from saltmarsh plant, Sesuvium portulacastrum L. Colloid. Surface B 2010, 79, 2488–2493. Search in Google Scholar
[197] Amin M, Anwar F, Janjua MRSA, Iqbal MA, Rashid U. Green synthesis of silver nanoparticles through reduction with Solanum xanthocarpum L. berry extract, characterization, antimicrobial and urease inhibitory activities against Helicobacter pylori. Int. J. Mol. Sci. 2012, 13, 9923–9941. Search in Google Scholar
[198] Njagi EC, Huang H, Stafford L, Genuino H, Galindo MH, Collins BJ, Hoag EG, Suib LS. Biosynthesis of iron and silver nanoparticles at room temperature using aqueous sorghum bran extracts. Langmuir 2011, 27, 264–271. Search in Google Scholar
[199] Petla RK, Vivekanandhan S, Misra M, Mohanty AK, Satyanarayana N. Soybean glycine max leaf extract based green synthesis of palladium nanoparticles. J. Biomater. Nanobiotech. 2012, 3, 14–19. Search in Google Scholar
[200] Mondal S, Roy N, Laskar RA, Sk I, Basu S, Mandal D, Begum NA. Biogenic synthesis of Ag, Au and bimetallic Au/Ag alloy nanoparticles using aqueous extract of mahogany Swietenia mahogani JACQ. leaves. Colloid. Surface B 2011, 82, 497–504. Search in Google Scholar
[201] Deshpande R, Bedre DM, Basavaraja S, Sawle B, Manjunath SY, Venkataraman A. Rapid biosynthesis of irregular shaped gold nanoparticles from macerated aqueous extracellular dried clove buds Syzygium aromaticum solution. Colloid. Surface B 2010, 79, 235–240. Search in Google Scholar
[202] Ankamwar B. Biosynthesis of gold nanoparticles (green-gold) using leaf extract of Terminalia catappa. E-J. Chem. 2010, 7, 1334–1339. Search in Google Scholar
[203] Geethalakshmi R, Sarada DVL. Synthesis of plantmediated silver nanoparticles using Trianthema decandra extract and evaluation of their anti microbial activities. Int. J. Eng. Sci. Technol. 2010, 2, 970–975. Search in Google Scholar
[204] Gopalakrishnan K, Ramesh C, Ragunathan V, Thamilselvan M. Antibacterial activity of copperoxide nanoparticles on E. coli synthesized from Tridax procumbens leaf extract and surface coating with polyaniline. Dig. J. Nanomater. Bios. 2012, 7, 833–839. Search in Google Scholar
[205] Pavani KV, Swati T, Snehika V, Sravya K, Sirisha M. Phytofabrication of lead nanoparticles using grape skin extract. Int. J. Eng. Sci. Tech. 2012, 4, 3376–3380. Search in Google Scholar
[206] Singh C, Sharma V, Naik KRP, Khandelwal V, Singh H. A green biogenic approach for synthesis of gold and silver nanoparticles using Zingiber officinale. Dig. J. Nanomater. Bios. 2011, 6, 535–542. Search in Google Scholar
[207] Dhuper S, Panda D, Nayak PL. Green synthesis and characterization of zero valent iron nanoparticles from the leaf extract of Mangifera indica. Nano Trends, A J. Nanotechnology and Its Applications 2012, 13, 16–22. Search in Google Scholar
[208] Pattanayak M, Mohapatra D, Nayak PL. Green synthesis and characterization of zero valent iron nanoparticles from the leaf extract of Syzygium aromaticum clove. Middle-E. J. Sci. Res. 2013, 18, 623–626. Search in Google Scholar
[209] Naseem T, Farrukh MA. Antibacterial activity of green synthesis of iron nanoparticles using Lawsonia inermis and Gardenia Jasminoides leaves extract. J. Chem. 2015, 7pages. Search in Google Scholar
[210] Weng X, Huang L, Chen Z, Megharaj M, Naidu R. Synthesis of iron-based nanoparticles by green tea extract and their degradation of malachite. Ind. Crop. Prod. 2013, 51, 342–347. Search in Google Scholar
[211] Pattanayak M, Nayak PL. Ecofriendly green synthesis of iron nanoparticles from various plants and spices extract. Int. J. Pl., An. Env. Sci. 2013, 3, 68–78. Search in Google Scholar
[212] Mohanraj S, Kodhaiyolii S, Rengasamy M, Pugalenthi V. Green synthesized iron oxide nanoparticles effect on fermentative hydrogen production by Clostridium acetobutylicum. Appl. Biochem. Biotech. 2014, 173, 318–331. Search in Google Scholar
[213] Makarov VV, Makarova SS, Love AJ, Sinitsyna OV, Dudnik AO, Yaminsky IV, Taliansky ME, Kalinina NO. Biosynthesis of stable iron oxide nanoparticles in aqueous extracts of Hordeum vulgare and Rumex acetosa plants. Langmuir 2014, 30, 5982–5988. Search in Google Scholar
[214] AbdelHamid AA, Al-Ghobashy MA, Fawzy M, Mohamed MB, Abdel Mottaleb MMSA. Phytosynthesis of Au, Ag, and Au-Ag bimetallic nanoparticles using aqueous extract of sago pondweed Potamogeton pectinatus L. ACS Sustainable Chem. Eng. 2013, 1, 1520–1529. Search in Google Scholar
[215] Kulkarni AA, Bhanage BM. Ag@AgCl nanomaterial synthesis using sugar cane juice and its application in degradation of azo dyes. ACS Sustainable Chem. Eng. 2014, 2, 1007–1013. Search in Google Scholar
[216] Patel VK, Bhattacharya S. High-performance nanothermite composites based on aloevera-directed CuO nanorods. ACS Appl. Mater. Interfaces. 2013, 5, 13364–13374. Search in Google Scholar
[217] Wang Z, Fang C, Megharaj M. Characterization of iron-polyphenol nanoparticles synthesized by three plant extracts and their fenton oxidation of azo dye. ACS Sustainable Chem. Eng. 2014, 2, 1022–1025. Search in Google Scholar
[218] Ashokkumar S, Ravi S, Velmuruganb S. Green synthesis of silver nanoparticles from Gloriosa superba L. leaf extract and their catalytic activity. Spectrochim. Acta A 2013, 115, 388–392. Search in Google Scholar
[219] Jagtap UB, Bapat VA. Biosynthesis, characterization and antibacterial activity of silver nanoparticles by aqueous Annona squamosa L. leaf extract at room temperature. J. Plant Biochem. Biot. 2013, 22, 434–440. Search in Google Scholar
[220] Haldar KM, Haldar B, Chandra G. Fabrication, characterization and mosquito larvicidal bioassay of silver nanoparticles synthesized from aqueous fruit extract of putranjiva, Drypetes roxburghii (Wall). Parasitol. Res. 2013, 112, 1451–1459. Search in Google Scholar
[221] Jha AK, Prasad K, Prasad K, Kulkarni AR. Plant system: nature’s nanofactory. Colloid. Surface B 2009, 73, 219–223. Search in Google Scholar
[222] Subhankari I, Nayak PL. Synthesis of copper nanoparticles using Syzygium aromaticum (cloves) aqueous extract by using green chemistry. World J. Nanosci. Nanotech. 2013, 2, 14–17. Search in Google Scholar
[223] Logeswari P, Silambarasan S, Abraham J. Synthesis of silver nanoparticles using plants extract and analysis of their antimicrobial property. Journal of Saudi Chemical Society 2015, 19, 311–317. Search in Google Scholar
[224] Jayaseelan C, Ramkumar R, Rahuman AA, Perumal P. Green synthesis of gold nanoparticles using seed aqueous extract of Abelmoschus esculentus and its antifungal activity. Ind. Crop. Prod. 2013, 45, 423–429. Search in Google Scholar
[225] Priya MM, Selvia KB, Paul JJA. Green synthesis of silver nanoparticles from the leaf extracts of Euphorbia hirta and Nerium indicum. Dig. J. Nanomater. Bios. 2011, 6, 869–877. Search in Google Scholar
[226] Das RK, Gogoi N, Bora U. Green synthesis of gold nanoparticles using Nyctanthes arbortristis flower extract. Bioprocess. Biosyst. Eng. 2011, 34, 615–619. Search in Google Scholar
[227] Forough M, Farhadi K. Biological and green synthesis of silver nanoparticles. Turkish J. Eng. Environ. Sci. 2010, 34, 281–287. Search in Google Scholar
[228] Raghunandan D, Basavaraja S, Mahesh B, Balaji S, Manjunath S, Venkataraman A. Biosynthesis of stable polyshaped gold nanoparticles from microwave-exposed aqueous extracellular anti-malignant guava Psidium guajava leaf extract. Nanobiotechnol. 2009, 5, 34–41. Search in Google Scholar
[229] Venkateswarlu S, Rao YS, Balaji T, Prathima B, Jyothi NVV. Biogenic synthesis of Fe3O4 magnetic nanoparticles using plantain peel extract. Mater. Lett. 2013, 100, 241–244. Search in Google Scholar
[230] Bankar A, Joshi B, Ravi Kumar A, Zinjarde S. Banana peel extract mediated novel route for the synthesis of palladium nanoparticles. Mater. Lett. 2010, 64, 1951–1953. Search in Google Scholar
[231] Venkateswarlu S, Natesh Kumar B, Prathima B, Anitha K, Jyothi NVV. A novel green synthesis of Fe3O4-Ag core shell recyclable nanoparticles using Vitis vinifera stem extract and its enhanced antibacterial performance. Physica B: Condensed Matter. 2015, 457, 30–35. Search in Google Scholar
[232] Nasrollahzadeh M, Sajadi SM, Rostami-Vartooni A, Khalaj M. Green synthesis of Pd/Fe3O4 nanoparticles using Euphorbia condylocarpa M. bieb root extract and their catalytic applications as magnetically recoverable and stable recyclable catalysts for the phosphine-free Sonogashira and Suzuki coupling reactions. J. Mol. Catal. A: Chem. 2015, 396, 31–39. Search in Google Scholar
[233] Otari SV, Patil RM, Ghosh SJ, Pawar SH. Green phytosynthesis of silver nanoparticles using aqueous extract of Manilkara zapota L. seeds and its inhibitory action against Candida species. Mater. Lett. 2014, 116, 367–369. Search in Google Scholar
[234] Ashokkumar S, Ravi S, Kathiravan V, Velmurugan S. Synthesis, characterization and catalytic activity of silver nanoparticles using Tribulus terrestris leaf extract. Spectrochim. Acta A 2014, 121, 88–93. Search in Google Scholar
[235] Seralathan J, Stevenson P, Subramaniam S, Raghavan R, Pemaiah B, Sivasubramanian A, Veerappan A. Spectroscopy investigation on chemo-catalytic, free radical scavenging and bactericidal properties of biogenic silver nanoparticles synthesized using Salicornia brachiata aqueous extract. Spectrochim. Acta A 2014, 118, 349–355. Search in Google Scholar
[236] Basavegowda N, Lee YR. Synthesis of silver nanoparticles using satsuma mandarin Citrus unshiu peel extract: a novel approach towards waste utilization. Mater. Lett. 2013, 109, 31–33. Search in Google Scholar
[237] Yallappa S, Manjanna J, Sindhe MA, Satyanarayan ND, Pramod SN, Nagaraja K. Microwave assisted rapid synthesis and biological evaluation of stable copper nanoparticles using T. arjuna bark extract. Spectrochim. Acta A 2013, 110, 108–115. Search in Google Scholar
[238] Yudha SS, Notriawan D, Angasa E, Suharto TE, Hendri J, Nishina Y. Green synthesis of silver nanoparticles using aqueous rinds extract of Brucea javanica L. Merr at ambient temperature. Mater. Lett. 2013, 97, 181–183. Search in Google Scholar
[239] Edison TJ, Sethuraman MG. Biogenic robust synthesis of silver nanoparticles using Punica granatum peel and its application as a green catalyst for the reduction of an anthropogenic pollutant 4-nitrophenol. Spectrochim. Acta A 2013, 104, 262–264. Search in Google Scholar
[240] Gopinath V, Priyadarshini S, Meera Priyadharsshini N, Pandian K, Velusamy P. Biogenic synthesis of antibacterial silver chloride nanoparticles using leaf extracts of Cissus quadrangularis Linn. Mater. Lett. 2013, 91, 224–227. Search in Google Scholar
[241] Cai Y, Shen Y, Xie A, Li S, Wang X. Green synthesis of soya bean sprouts-mediated superparamagnetic Fe3O4 nanoparticles. J. Magn. Magn. Mater. 2010, 322, 2938–2943. Search in Google Scholar
[242] Kumar B, Smita K, Cumbal L, Debut A. Biogenic synthesis of iron oxide nanoparticles for 2-arylbenzimidazole fabrication. J. Saudi Chem. Soc. 2014, 18, 364–369. Search in Google Scholar
[243] Basavegowda N, Somai Magar KB, Mishra K, Lee YR. Green fabrication of ferromagnetic Fe3O4 nanoparticles and their novel catalytic applications for the synthesis of biologically interesting benzoxazinone and benzthioxazinone derivatives. N. J. Chem. 2014, 38, 5415–5420. Search in Google Scholar
[244] Bonde S. A biogenic approach for green synthesis of silver nanoparticles using extract of Foeniculum vulgare and its activity against Staphylococcus aureus and Escherichia coli. Nusantara Biosci. 2011, 3, 59–63. Search in Google Scholar
[245] Shekhawat MS, Kannan N, Manokari M. Biogenesis of silver nanoparticles using leaf extract of Turnera ulmifolia Linn. and screening of their antimicrobial activity. J. Ecobiotechnol. 2012, 4, 54–57. Search in Google Scholar
[246] Govindaraju K, Tamilselvan S, Kiruthiga V, Singaravelu G. Biogenic silver nanoparticles by Solanum torvum and their promising antimicrobial activity. J. Biopestic . 2010, 3, 394–399. Search in Google Scholar
[247] Basu S, Maji P, Ganguly J. Rapid green synthesis of silver nanoparticles by aqueous extract of seeds of Nyctanthes arbor-tristis . Appl. Nanosci. 2015, DOI 10.1007/s13204-015-0407-9. Search in Google Scholar
[248] Sasikala A, Savithramma N. Biological synthesis of silver nanoparticles from Cochlospermum religiosum and their antibacterial efficacy. J. Pharm Sci. Res. 2012, 4, 1836–1839. Search in Google Scholar
[249] Yogalakshmi M, Sumalatha D, Madhan R. Biosynthesis of silver nanoparticles using xtracts of Polyalthia longifolia leaves and their antimicrobial properties. J. Biological Inform. Sci. 2012, 1, 21–24. Search in Google Scholar
[250] Sivakumar J, Premkumar C, Santhanam P, Saraswathi N. Biosynthesis of silver nanoparticles using Calotropis gigantean leaf. Afr. J. Basic Appl. Sci. 2011, 3, 265–270. Search in Google Scholar
[251] Shukla VK, Singh RP, Pandey AC. Black pepper assisted biomimetic synthesis of silver nanoparticles. J. Alloys Compd. 2010, 507, L13–L16. Search in Google Scholar
[252] Zargar M, Hamid AA, Bakar FA, Shamsudin MN. Green synthesis and antibacterialeffect of silver nanoparticles using Vitex negundo L. Molecules 2011, 16, 6667–6676. Search in Google Scholar
[253] Awwad AM, Salem NM. Green synthesis of silver nanoparticles by mulberry leaves extract. Nanosci. Nanotechnol . 2012, 2, 125–128. Search in Google Scholar
[254] Korbekandi H, Asghari G, Jalayer SS, Jalayer MS, Bandegani M. Nanosilver particle production using Juglans Regia L. (walnut) leaf extract. J. Nat. Pharm. Prod. 2013, 8, 20–26. Search in Google Scholar
[255] Kulkarni AP, Srivastava AA, Harpale PM, Zunjarrao RS. Plant mediated synthesis of silver nanoparticles – tapping the unexploited sources. J. Nat. Prod. Plant Resour . 2011, 1, 100–107. Search in Google Scholar
[256] Bali R, Razak N, Lumb A, Harris AT. The synthesis of metal nanoparticles inside live plants. International Conference on Nanoscience and Nanotechnology, IEEE Xplore . 2006, DOI 10.1109/ICONN.2006.340592 2006. Search in Google Scholar
[257] Parashar V, Parashar R, Sharma B, Pandey AC. Parthenium leaf extract mediated synthesis of silver nanoparticles, a novel approach towards weed utilization. Dig. J. Nanomater. Bios. 2009, 4, 45–50. Search in Google Scholar
[258] Roy N, Barik A. Green Synthesis of silver nanoparticles from the unexploited weed resources. Int. J. Nanotechnol. App. 2010, 42, 95–101. Search in Google Scholar
©2015 by De Gruyter
- X / Twitter
Supplementary Materials
Please login or register with De Gruyter to order this product.
Journal and Issue
Articles in the same issue.
A review on the green synthesis of nanoparticles, their biological applications, and photocatalytic efficiency against environmental toxins
- Review Article
- Published: 12 May 2023
- Volume 30 , pages 69796–69823, ( 2023 )
Cite this article
- Azad Qayoom Malik ORCID: orcid.org/0000-0003-4858-4351 1 ,
- Tahir ul Gani Mir 2 ,
- Deepak Kumar 1 ,
- Irtiqa Ashraf Mir 1 ,
- Adfar Rashid 1 ,
- Mehnaz Ayoub 1 &
- Saurabh Shukla 2
1146 Accesses
10 Citations
Explore all metrics
Green synthesis of nanoparticles (NPs) using plant materials and microorganisms has evolved as a sustainable alternative to conventional techniques that rely on toxic chemicals. Recently, green-synthesized eco-friendly NPs have attracted interest for their potential use in various biological applications. Several studies have demonstrated that green-synthesized NPs are beneficial in multiple medicinal applications, including cancer treatment, targeted drug delivery, and wound healing. Additionally, due to their photodegradation activity, green-synthesized NPs are a promising tool in environmental remediation. Photodegradation is a process that uses light and a photocatalyst to turn a pollutant into a harmless product. Green NPs have been found efficient in degrading pollutants such as dyes, herbicides, and heavy metals. The use of microbes and flora in green synthesis technology for nanoparticle synthesis is biologically safe, cost-effective, and eco-friendly. Plants and microbes can now use and accumulate inorganic metallic ions in the environment. Various NPs have been synthesized via the bio-reduction of biological entities or their extracts. There are several biological and environmental uses for biologically synthesized metallic NPs, such as photocatalysis, adsorption, and water purification. Since the last decade, the green synthesis of NPs has gained significant interest in the scientific community. Therefore, there is a need for a review that serves as a one-stop resource that points to relevant and recent studies on the green synthesis of NPs and their biological and photocatalytic efficiency. This review focuses on the green fabrication of NPs utilizing diverse biological systems and their applications in biological and photodegradation processes.
Graphical Abstract
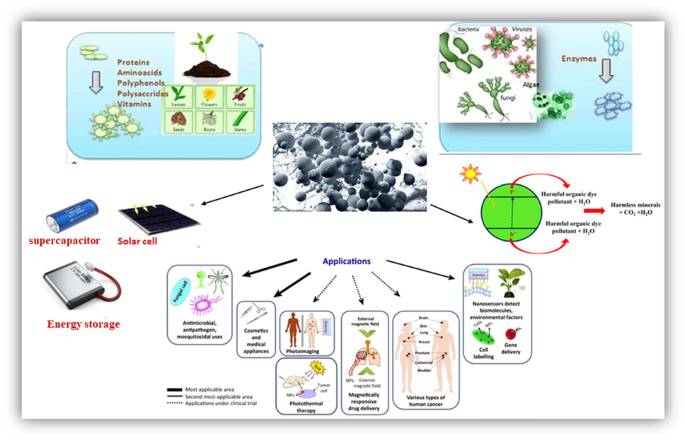
This is a preview of subscription content, log in via an institution to check access.
Access this article
Price includes VAT (Russian Federation)
Instant access to the full article PDF.
Rent this article via DeepDyve
Institutional subscriptions
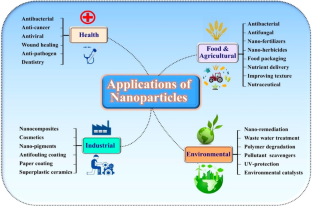
Similar content being viewed by others
Green Synthesis of Nanoparticles and Their Application for Sustainable Environment
Green Synthesis of Metal Oxide Nanomaterials and Photocatalytic Degradation of Toxic Dyes
Data availability
Not applicable.
Adeyemi JO, Oriola AO, Onwudiwe DC, Oyedeji AO (2022) Plant extracts mediated metal-based nanoparticles: synthesis and biological applications. Biomolecules 12(5):627. https://doi.org/10.3390/biom12050627
Article CAS Google Scholar
Adibkia K, Alaei-Beirami M, Barzegar-Jalali M, Mohammadi G, Ardestani MS (2012) Evaluation and optimization of factors affecting novel diclofenac sodium-eudragit RS100 nanoparticles. Afr J Pharm Pharmacol 6(12), 941–947. https://www.cabdirect.org/globalhealth/abstract/20123155403
Ahmad N (2012) Green synthesis of silver nanoparticles using extracts of Ananas comosus. Green Sustain Chem 02:141–147. https://doi.org/10.4236/gsc.2012.24020
Ahmed A-A, Hamzah H, Maaroof M, Suood A (2018) Analyzing formation of silver nanoparticles from the filamentous fungus Fusarium oxysporum and their antimicrobial activity. Turk J Biol 42. https://doi.org/10.3906/biy-1710-2
Aj H, Yj K (2011) “Nanoantibiotics”: a new paradigm for treating infectious diseases using nanomaterials in the antibiotics resistant era. J Control Release : Official Journal of the Controlled Release Society 156(2). https://doi.org/10.1016/j.jconrel.2011.07.002
Alam T, Khan R, Ali A, Sher H, Ullah Z, Ali M (2019) Biogenic synthesis of iron oxide nanoparticles via Skimmia laureola and their antibacterial efficacy against bacterial wilt pathogen Ralstonia solanacearum. Mater Sci Eng, C 98:101–108. https://doi.org/10.1016/j.msec.2018.12.117
Alavi M, Karimi N (2018) Characterization, antibacterial, total antioxidant, scavenging, reducing power and ion chelating activities of green synthesized silver, copper and titanium dioxide nanoparticles using Artemisia haussknechtii leaf extract. Artif Cells, Nanomed Biotechnol 46(8):2066–2081. https://doi.org/10.1080/21691401.2017.1408121
Al-Hakkani MF (2020) Biogenic copper nanoparticles and their applications: a review. SN Appl Sci 2(3):505. https://doi.org/10.1007/s42452-020-2279-1
Ali MA, Ahmed T, Wu W, Hossain A, Hafeez R, Islam Masum MM, Wang Y, An Q, Sun G, Li B (2020) Advancements in plant and microbe-based synthesis of metallic nanoparticles and their antimicrobial activity against plant pathogens. Nanomaterials, 10(6), Article 6. https://doi.org/10.3390/nano10061146
Armendariz V, Herrera I, Peralta-Videa JR, Jose-Yacaman M, Troiani H, Santiago P, Gardea-Torresdey JL (2004) Size controlled gold nanoparticle formation by Avena sativa biomass: Use of plants in nanobiotechnology. J Nanopart Res 6(4):377–382. https://doi.org/10.1007/s11051-004-0741-4
Asimuddin M, Shaik MR, Adil SF, Siddiqui MRH, Alwarthan A, Jamil K, Khan M (2020) Azadirachta indica based biosynthesis of silver nanoparticles and evaluation of their antibacterial and cytotoxic effects. J King Saud Univ - Sci 32(1):648–656. https://doi.org/10.1016/j.jksus.2018.09.014
Article Google Scholar
Ayala V, Herrera AP, Latorre-Esteves M, Torres-Lugo M, Rinaldi C (2013) Effect of surface charge on the colloidal stability and in vitro uptake of carboxymethyl dextran-coated iron oxide nanoparticles. J Nanopart Res 15(8):1874
Baco-Carles V, Datas L, Tailhades P (2011) Copper nanoparticles prepared from oxalic precursors. ISRN Nanotechnol 2011:1–7. https://doi.org/10.5402/2011/729594
Badawy AME, Luxton TP, Silva RG, Scheckel KG, Suidan MT, Tolaymat TM (2010) Impact of environmental conditions (pH, ionic strength, and electrolyte type) on the surface charge and aggregation of silver nanoparticles suspensions. Environ Sci Technol 44(4):1260–1266
Baer D (2011) Surface characterization of nanoparticles: critical needs and significant challenges. J Surf Anal (online) 17:163–169
Baker S, Rakshith D, Kavitha KS, Santosh P, Kavitha HU, Rao Y, Satish S (2013) Plants: emerging as nanofactories towards facile route in synthesis of nanoparticles. Bioimpacts 3(3):111–117. https://doi.org/10.5681/bi.2013.012
Banu AN, Balasubramanian C (2014) Optimization and synthesis of silver nanoparticles using Isaria fumosorosea against human vector mosquitoes. Parasitol Res 113(10):3843–3851. https://doi.org/10.1007/s00436-014-4052-0
Bar H, Bhui D, Sahoo G, Sarkar P, Pyne S, Misra A (2009) Green synthesis of silver nanoparticles using seed extract of Jatropha curcas. Colloids SurfA: Physicochem Eng Aspects 348:212–216. https://doi.org/10.1016/j.colsurfa.2009.07.021
Barzinjy AA, Azeez HH (2020) Green synthesis and characterization of zinc oxide nanoparticles using Eucalyptus globulus Labill. Leaf extract and zinc nitrate hexahydrate salt. SN Appl Sci 2(5):991. https://doi.org/10.1007/s42452-020-2813-1
Basak S, Venkatram R, Singhal RS (2022) Recent advances in the application of molecularly imprinted polymers (MIPs) in food analysis. Food Control 139:109074. https://doi.org/10.1016/j.foodcont.2022.109074
Bhardwaj K, Dhanjal DS, Sharma A, Nepovimova E, Kalia A, Thakur S, Bhardwaj S, Chopra C, Singh R, Verma R, Kumar D, Bhardwaj P, Kuča K (2020) Conifer-derived metallic nanoparticles: green synthesis and biological applications. Int J Mol Sci 21(23), Article 23. https://doi.org/10.3390/ijms21239028
Bhattarai B, Zaker Y, Bigioni TP (2018) Green synthesis of gold and silver nanoparticles: challenges and opportunities. Curr Opin Green Sustain Chem 12:91–100
Bhosale MG, Sutar RS, Londhe SS, Patil MK (2022) Sol–gel method synthesized Ce-doped TiO2 visible light photocatalyst for degradation of organic pollutants. Appl Organomet Chem 36(4):e6586. https://doi.org/10.1002/aoc.6586
Bhuiyan MdSH, Miah MY, Paul SC, Aka TD, Saha O, Rahaman MdM, Sharif MdJI, Habiba O, Ashaduzzaman Md (2020) Green synthesis of iron oxide nanoparticle using Carica papaya leaf extract: application for photocatalytic degradation of remazol yellow RR dye and antibacterial activity. Heliyon 6(8):e04603. https://doi.org/10.1016/j.heliyon.2020.e04603
Bibi I, Kamal S, Ahmed A, Iqbal M, Nouren S, Jilani K, Nazar N, Amir M, Abbas A, Ata S, Majid F (2017a) Nickel nanoparticle synthesis using Camellia Sinensis as reducing and capping agent: growth mechanism and photo-catalytic activity evaluation. Int J Biol Macromol 103:783–790. https://doi.org/10.1016/j.ijbiomac.2017.05.023
Bibi I, Nazar N, Iqbal M, Kamal S, Nawaz H, Nouren S, Safa Y, Jilani K, Sultan M, Ata S, Rehman F, Abbas M (2017b) Green and eco-friendly synthesis of cobalt-oxide nanoparticle: characterization and photo-catalytic activity. Adv Powder Technol 28(9):2035–2043. https://doi.org/10.1016/j.apt.2017.05.008
Buzea C, Pacheco II, Robbie K (2007) Nanomaterials and nanoparticles: sources and toxicity. Biointerphases 2(4):MR17-71. https://doi.org/10.1116/1.2815690
Cao Y, Zhou G, Zhou R, Wang C, Chi B, Wang Y, Hua C, Qiu J, Jin Y, Wu S (2020) Green synthesis of reusable multifunctional γ-Fe2O3/bentonite modified by doped TiO2 hollow spherical nanocomposite for removal of BPA. Sci Total Environ 708:134669. https://doi.org/10.1016/j.scitotenv.2019.134669
Castillo-Henríquez L, Alfaro-Aguilar K, Ugalde-Álvarez J, Vega-Fernández L, Montes de Oca-Vásquez G, Vega-Baudrit JR (2020) Green synthesis of gold and silver nanoparticles from plant extracts and their possible applications as antimicrobial agents in the agricultural area. Nanomaterials 10(9):1763. https://doi.org/10.3390/nano10091763
Castro-Longoria E, Moreno-Velázquez S, Vilchis-Nestor A, Arenas E, Avalos-Borja M (2012) Production of platinum nanoparticles and nanoaggregates using Neurospora crassa. J Microbiol Biotechnol 22:1000–1004. https://doi.org/10.4014/jmb.1110.10085
Chahardoli A, Karimi N, Sadeghi F, Fattahi A (2018) Green approach for synthesis of gold nanoparticles from Nigella arvensis leaf extract and evaluation of their antibacterial, antioxidant, cytotoxicity and catalytic activities. Artif Cells, Nanomed Biotechnol 46(3):579–588. https://doi.org/10.1080/21691401.2017.1332634
Chakraborty S, Basak B, Dutta S, Bhunia B, Dey A (2013) Decolorization and biodegradation of congo red dye by a novel white rot fungus Alternaria alternata CMERI F6. Bioresour Technol 147. https://doi.org/10.1016/j.biortech.2013.08.117
Chandra H, Kumari P, Bontempi E, Yadav S (2020) Medicinal plants: treasure trove for green synthesis of metallic nanoparticles and their biomedical applications. Biocatal Agric Biotechnol 24:101518
Chandran S, Chaudhary M, Pasricha R, Ahmad A, Sastry M (2006) Synthesis of gold nanotriangles and silver nanoparticles using aloe vera plant extract. Biotechnol Prog 22:577–583. https://doi.org/10.1021/bp0501423
Chang W, Liu S, Qileng A, Liu W, Liu Y (2018) In-situ synthesis of monodispersed Au nanoparticles on eggshell membrane by the extract of Lagerstroemia speciosa leaves for the catalytic reduction of 4-nitrophenol. Mater Res Express 6(1):015002. https://doi.org/10.1088/2053-1591/aae2f0
Chang B-Y, Koo B-S, Kim S-Y (2021) Pharmacological activities for Morus alba L., focusing on the immunostimulatory property from the fruit aqueous extract. Foods 10(8):1966. https://doi.org/10.3390/foods10081966
Chaurasia PK, Bharati SL, Yadava S (2022) Nano-reduction of gold and silver ions: a perspective on the fate of microbial laccases as potential biocatalysts in the synthesis of metals (gold and silver) nano-particles. Curr Res Microb Sci 3:100098. https://doi.org/10.1016/j.crmicr.2021.100098
Chen S, Kucernak A (2004) Electrocatalysis under conditions of high mass transport rate: oxygen reduction on single submicrometer-sized Pt particles supported on carbon. J Phys Chem B 108(10):3262–3276
Chidambaram J, Rahuman A, Roopan S, Kirthi V, Venkatesan J, Kim S-K, Iyappan M, Siva C (2013) Biological approach to synthesize TiO2 nanoparticles using Aeromonas hydrophila and its antibacterial activity. Spectrochimica Acta Part A: Molecular and Biomol Spectrosc 107C. https://doi.org/10.1016/j.saa.2012.12.083
Chopra H, Bibi S, Singh I, Hasan MM, Khan MS, Yousafi Q, Baig AA, Rahman MM, Islam F, Emran TB, Cavalu S (2022) Green metallic nanoparticles: biosynthesis to applications. Front Bioeng Biotechnol. https://doi.org/10.3389/fbioe.2022.874742
Danish MSS, Estrella-Pajulas LL, Alemaida IM, Grilli ML, Mikhaylov A, Senjyu T (2022) Green synthesis of silver oxide nanoparticles for photocatalytic environmental remediation and biomedical applications. Metals, 12(5), Article 5. https://doi.org/10.3390/met12050769
Darroudi M, Ahmad M, Zamiri R, Khorsand Zak A, Abdullah A, Ibrahim N (2011) Time-dependent effect in green synthesis of silver nanoparticles. Int J Nanomed 6. https://doi.org/10.2147/IJN.S17669
Das RK, Gogoi N, Bora U (2011) Green synthesis of gold nanoparticles using Nyctanthes arbortristis flower extract. Bioprocess Biosyst Eng 34(5):615–619. https://doi.org/10.1007/s00449-010-0510-y
Das C, Sen S, Singh T, Ghosh T, Paul SS, Kim TW, Jeon S, Maiti DK, Im J, Biswas G (2020) Green synthesis, characterization and application of natural product coated magnetite nanoparticles for wastewater treatment. Nanomaterials, 10(8), Article 8. https://doi.org/10.3390/nano10081615
Dash SR, Kundu CN (2020) Promising opportunities and potential risk of nanoparticle on the society. IET Nanobiotechnol 14(4):253–260. https://doi.org/10.1049/iet-nbt.2019.0303
de Vinicius Oliveira Brisola Maciel M, da Rosa Almeida A, Machado MH, Elias WC, Gonçalves da Rosa C, Teixeira GL, Noronha CM, Bertoldi FC, Nunes MR, Dutra de Armas R, Manique Barreto PL (2020) Green synthesis, characteristics and antimicrobial activity of silver nanoparticles mediated by essential oils as reducing agents. Biocatal Agric Biotechnol 28:101746. https://doi.org/10.1016/j.bcab.2020.101746
Dikshit PK, Kumar J, Das A, Sadhu S, Sharma S, Singh S, Gupta P, Kim BS (2021) Green synthesis of metallic nanoparticles: applications and limitations. Catalysts 11:1–37. https://doi.org/10.3390/catal11080902
Dil EA, Ghaedi M, Asfaram A (2017) The performance of nanorods material as adsorbent for removal of azo dyes and heavy metal ions: application of ultrasound wave, optimization and modeling. Ultrason Sonochem 34:792–802. https://doi.org/10.1016/j.ultsonch.2016.07.015
Drummer S, Madzimbamuto T, Chowdhury M (2021) Green synthesis of transition-metal nanoparticles and their oxides: a review. Materials, 14(11), Article 11. https://doi.org/10.3390/ma14112700
Dzimitrowicz A, Berent S, Motyka A, Jamroz P, Kurcbach K, Sledz W, Pohl P (2019) Comparison of the characteristics of gold nanoparticles synthesized using aqueous plant extracts and natural plant essential oils of Eucalyptus globulus and Rosmarinus officinalis. Arab J Chem 12(8):4795–4805. https://doi.org/10.1016/j.arabjc.2016.09.007
Edison TJI, Sethuraman MG (2012) Instant green synthesis of silver nanoparticles using Terminalia chebula fruit extract and evaluation of their catalytic activity on reduction of methylene blue. Process Biochem 47(9):1351–1357. https://doi.org/10.1016/j.procbio.2012.04.025
Elamawi RM, Al-Harbi RE, Hendi AA (2018) Biosynthesis and characterization of silver nanoparticles using Trichoderma longibrachiatum and their effect on phytopathogenic fungi. Egyptian J Biol Pest Control 28(1):28. https://doi.org/10.1186/s41938-018-0028-1
Elbeshehy EKF, Elazzazy AM, Aggelis G (2015) Silver nanoparticles synthesis mediated by new isolates of Bacillus spp., nanoparticle characterization and their activity against Bean Yellow Mosaic Virus and human pathogens. Front Microbiol 6:453. https://doi.org/10.3389/fmicb.2015.00453
Eldomany E, Essam TM, Ahmed AE, Farghali A (2018) Biosynthesis physico-chemical optimization of gold nanoparticles as anti-cancer and synergetic antimicrobial activity using Pleurotus ostreatus fungus. J Appl Pharm Sci 8:119–128. https://doi.org/10.7324/JAPS.2018.8516
El-Sayed MEA (2020) Nanoadsorbents for water and wastewater remediation. Sci Total Environ 739:139903. https://doi.org/10.1016/j.scitotenv.2020.139903
Fouda A, El-Din Hassan S, Salem SS, Shaheen TI (2018) In-vitro cytotoxicity, antibacterial, and UV protection properties of the biosynthesized zinc oxide nanoparticles for medical textile applications. Microb Pathog 125:252–261. https://doi.org/10.1016/j.micpath.2018.09.030
Fujiwara M, Imura T (2015) Photo induced membrane separation for water purification and desalination using azobenzene modified anodized alumina membranes. ACS Nano 9(6):5705–5712. https://doi.org/10.1021/nn505970n
Gahlawat G, Roy Choudhury A (2019) A review on the biosynthesis of metal and metal salt nanoparticles by microbes. RSC Adv 9(23):12944–12967. https://doi.org/10.1039/C8RA10483B
Gao G, Ze Y, Li B, Zhao X, Zhang T, Sheng L, Hu R, Gui S, Sang X, Sun Q, Cheng J, Cheng Z, Wang L, Tang M, Hong F (2012) Ovarian dysfunction and gene-expressed characteristics of female mice caused by long-term exposure to titanium dioxide nanoparticles. J Hazard Mater 243:19–27. https://doi.org/10.1016/j.jhazmat.2012.08.049
Ghosh S, Ahmad R, Zeyaullah Md, Khare SK (2021) Microbial nano-factories: synthesis and biomedical applications. Front Chem 9:626834. https://doi.org/10.3389/fchem.2021.626834
Ghotekar S, Dabhane H, Pansambal S, Oza R, Tambade P, Medhane V (2020) A review on biomimetic synthesis of Ag2O Nanoparticles using plant extract, characterization and its recent applications. Adv J Chem-Sect B, 2(3). https://doi.org/10.22034/ajcb.2020.107810
Gnanasekar S, Murugaraj J, Balakrishnan D, Krishnamoorthy V, Jha PK, Seetharaman PK, Vilwanathan R, Sivaperumal S (2017) Antibacterial and cytotoxicity effects of biogenic palladium nanoparticles synthesized using fruit extract of Couroupita guianensis Aubl. J Appl Biomed16. https://doi.org/10.1016/j.jab.2017.10.001
Gopalakrishnan K, Chandel M, Gupta V, Kaur K, Patel A, Kaur K, Kishore A, Prabhakar PK, Singh A, Prasad JS (2023) Valorisation of fruit peel bioactive into green synthesized silver nanoparticles to modify cellulose wrapper for shelf-life extension of packaged bread. Food Res Int 164:112321
Govindasamy S, Thirumarimurugan M, Muthukumaran C (2018) Green synthesis of ZnO nanoparticles using Tecoma castanifolia leaf extract: characterization and evaluation of its antioxidant, bactericidal and anticancer activities. Microchem J 145. https://doi.org/10.1016/j.microc.2018.11.022
Guan Z, Ying S, Ofoegbu PC, Clubb P, Rico C, He F, Hong J (2022) Green synthesis of nanoparticles: current developments and limitations. Environ Technol Innov 102336
Guerrini L, Alvarez-Puebla RA, Pazos-Perez N (2018) Surface modifications of nanoparticles for stability in biological fluids. Materials 11(7):1154
Gupta R, Xie H (2018) Nanoparticles in daily life: applications, toxicity and regulations. J Environ Pathol Toxicol Oncol 37. https://doi.org/10.1615/JEnvironPatholToxicolOncol.2018026009
Gupta K, Chundawat TS (2019) Bio-inspired synthesis of platinum nanoparticles from fungus Fusarium oxysporum: its characteristics, potential antimicrobial, antioxidant and photocatalytic activities. Mater Res Express 6(10):1050d6. https://doi.org/10.1088/2053-1591/ab4219
Habibullah G, Viktorova J, Ulbrich P, Ruml T (2022) Effect of the physicochemical changes in the antimicrobial durability of green synthesized silver nanoparticles during their long-term storage. RSC Adv 12(47):30386–30403
Hennebel T, Van Nevel S, Verschuere S, De Corte S, De Gusseme B, Cuvelier C, Fitts J, Lelie D, Boon N, Verstraete W (2011) Palladium nanoparticles produced by fermentatively cultivated bacteria as catalyst for diatrizoate removal with biogenic hydrogen. Appl Microbiol Biotechnol 91:1435–1445. https://doi.org/10.1007/s00253-011-3329-9
Hernández-Díaz JA, Garza-García JJ, Zamudio-Ojeda A, León-Morales JM, López-Velázquez JC, García-Morales S (2021) Plant-mediated synthesis of nanoparticles and their antimicrobial activity against phytopathogens. J Sci Food Agric 101(4):1270–1287. https://doi.org/10.1002/jsfa.10767
Ho PL, Chow KH, Yuen KY, Ng WS, Chau PY (1998) Comparison of a novel, inhibitor-potentiated disc-diffusion test with other methods for the detection of extended-spectrum beta-lactamases in Escherichia coli and Klebsiella pneumoniae. J Antimicrob Chemother 42(1):49–54. https://doi.org/10.1093/jac/42.1.49
Hosny M, Fawzy M, El-Badry YA, Hussein EE, Eltaweil AS (2022) Plant-assisted synthesis of gold nanoparticles for photocatalytic, anticancer, and antioxidant applications. J Saudi Chem Soc 26(2):101419. https://doi.org/10.1016/j.jscs.2022.101419
Huang J, Li Q, Sun D, Lu Y, Su Y, Yang X, Wang H, Wang Y, Shao W, He N, Hong J, Chen C (2007) Biosynthesis of silver and gold nanoparticles by novel sundried Cinnamomum camphora leaf. Nanotechnology 18(10):105104. https://doi.org/10.1088/0957-4484/18/10/105104
Huang H, Steiniger KA, Lambert TH (2022) Electrophotocatalysis: combining light and electricity to catalyze reactions. J Am Chem Soc 144(28):12567–12583
Ijaz I, Gilani E, Nazir A, Bukhari A (2020) Detail review on chemical, physical and green synthesis, classification, characterizations and applications of nanoparticles. Green Chem Lett Rev 13(3):223–245
Iqtedar M, Aslam M, Farrukh MA, Shahzad A, Abdullah R, Kaleem A (2019) Extracellular biosynthesis, characterization, optimization of silver nanoparticles (AgNPs) using Bacillus mojavensis BTCB15 and its antimicrobial activity against multidrug resistant pathogens. Prep Biochem Biotechnol 49:1–7. https://doi.org/10.1080/10826068.2018.1550654
Iravani S (2011) Green synthesis of metal nanoparticles using plants. Green Chem 13(10):2638–2650
Jafarzadeh S, Jafari SM (2021) Impact of metal nanoparticles on the mechanical, barrier, optical and thermal properties of biodegradable food packaging materials. Crit Rev Food Sci Nutr 61(16):2640–2658
Jalal M, Ansari MA, Alzohairy MA, Ali SG, Khan HM, Almatroudi A, Raees K (2018) Biosynthesis of silver nanoparticles from oropharyngeal Candida glabrata isolates and their antimicrobial activity against clinical strains of bacteria and fungi. Nanomaterials 8(8):586. https://doi.org/10.3390/nano8080586
Javed R, Zia M, Naz S, Aisida SO, Ain N, ul, & Ao, Q. (2020) Role of capping agents in the application of nanoparticles in biomedicine and environmental remediation: recent trends and future prospects. J Nanobiotechnol 18:1–15
Jenning V, Gohla SH (2001) Encapsulation of retinoids in solid lipid nanoparticles (SLN). J Microencapsul 18(2):149–158
Jiang W, Mashayekhi H, Xing B (2009) Bacterial toxicity comparison between nano- and micro-scaled oxide particles. (Environ Pollut Barking, EssexL 1987) 157(5):1619–1625. https://doi.org/10.1016/j.envpol.2008.12.025
Kahsay MH, RamaDevi D, Kumar YP, Mohan BS, Tadesse A, Battu G, Basavaiah K (2018) Synthesis of silver nanoparticles using aqueous extract of Dolichos lablab for reduction of 4-nitrophenol, antimicrobial and anticancer activities. OpenNano 3:28–37. https://doi.org/10.1016/j.onano.2018.04.001
Kalimuthu K, Suresh Babu R, Venkataraman D, Bilal M, Gurunathan S (2008) Biosynthesis of silver nanocrystals by Bacillus licheniformis. Colloids Surf B, Biointerfaces 65(1):150–153. https://doi.org/10.1016/j.colsurfb.2008.02.018
Kamran U, Bhatti HN, Iqbal M, Nazir A (2019) Green synthesis of metal nanoparticles and their applications in different fields: a review. Z Phys Chem 233(9):1325–1349. https://doi.org/10.1515/zpch-2018-1238
Kartha B, Thanikachalam K, Vijayakumar N, Alharbi NS, Kadaikunnan S, Khaled JM, Gopinath K, Govindarajan M (2022) Synthesis and characterization of Ce-doped TiO2 nanoparticles and their enhanced anticancer activity in Y79 retinoblastoma cancer cells. Green Process Synth 11(1):143–149. https://doi.org/10.1515/gps-2022-0011
Katta VKM, Dubey RS (2021) Green synthesis of silver nanoparticles using Tagetes erecta plant and investigation of their structural, optical, chemical and morphological properties. Mater Today: Proceedings 45:794–798. https://doi.org/10.1016/j.matpr.2020.02.809
Koul B, Poonia AK, Yadav D, Jin J-O (2021) Microbe-mediated biosynthesis of nanoparticles: applications and future prospects. Biomolecules 11(6):886. https://doi.org/10.3390/biom11060886
Kumar M, Mehta A, Mishra A, Singh J, Rawat M, Basu S (2018) Biosynthesis of tin oxide nanoparticles using Psidium Guajava leave extract for photocatalytic dye degradation under sunlight. Mater Lett 215:121–124. https://doi.org/10.1016/j.matlet.2017.12.074
Kuppusamy P, Yusoff MM, Maniam GP, Govindan N (2016) Biosynthesis of metallic nanoparticles using plant derivatives and their new avenues in pharmacological applications – an updated report. Saudi Pharm J 24(4):473–484. https://doi.org/10.1016/j.jsps.2014.11.013
Laokul P, Klinkaewnarong J, Phokha S, Seraphin S (2008) Indium oxide (In2O3) nanoparticles using aloe vera plant extract: synthesis and optical properties. Optoelectron Adv Mater Rapid Commun 2
Laouini SE, Bouafia A, Soldatov AV, Algarni H, Tedjani ML, Ali GAM, Barhoum A (2021) Green synthesized of Ag/Ag2O nanoparticles using aqueous leaves extracts of Phoenix dactylifera L. and their azo dye photodegradation. Membranes, 11(7), Article 7. https://doi.org/10.3390/membranes11070468
Levard C, Hotze EM, Lowry GV, Brown GE Jr (2012) Environmental transformations of silver nanoparticles: impact on stability and toxicity. Environ Sci Technol 46(13):6900–6914
Liang T, Qiu X, Ye X, Liu Y, Li Z, Tian B, Yan D (2020) Biosynthesis of selenium nanoparticles and their effect on changes in urinary nanocrystallites in calcium oxalate stone formation. 3 Biotech, 10. https://doi.org/10.1007/s13205-019-1999-7
Liu L, Corma A (2021) Structural transformations of solid electrocatalysts and photocatalysts. Nat Rev Chem 5(4):256–276
López-Miranda JL, Esparza R, Rosas G, Pérez R, Estévez-González M (2019) Catalytic and antibacterial properties of gold nanoparticles synthesized by a green approach for bioremediation applications. 3 Biotech 9(4):135. https://doi.org/10.1007/s13205-019-1666-z
Love AJ, Makarov VV, Sinitsyna OV, Shaw J, Yaminsky IV, Kalinina NO, Taliansky ME (2015) A genetically modified tobacco mosaic virus that can produce gold nanoparticles from a metal salt precursor. Front Plant Sci 6. https://doi.org/10.3389/fpls.2015.00984
Lukman AI, Gong B, Marjo CE, Roessner U, Harris AT (2011) Facile synthesis, stabilization, and anti-bacterial performance of discrete Ag nanoparticles using Medicago sativa seed exudates. J Colloid Interface Sci 353(2):433–444. https://doi.org/10.1016/j.jcis.2010.09.088
Lv Q, Zhang B, Xing X, Zhao Y, Cai R, Wang W, Gu Q (2018) Biosynthesis of copper nanoparticles using Shewanella loihica PV-4 with antibacterial activity: novel approach and mechanisms investigation. J Hazard Mater 347:141–149. https://doi.org/10.1016/j.jhazmat.2017.12.070
Lynch I, Cedervall T, Lundqvist M, Cabaleiro-Lago C, Linse S, Dawson KA (2007) The nanoparticle-protein complex as a biological entity; a complex fluids and surface science challenge for the 21st century. Adv Coll Interface Sci 134–135:167–174. https://doi.org/10.1016/j.cis.2007.04.021
Mahmoudi M, Sant S, Wang B, Laurent S, Sen T (2011) Superparamagnetic iron oxide nanoparticles (SPIONs): development, surface modification and applications in chemotherapy. Adv Drug Deliv Rev 63(1–2):24–46
Malik AQ, Singh H, Kumar A, Aepuru R, Kumar D, Mir ul TG, ul Ain Q, Bhat AA, Mubayi A (2022a) An overview on magnetic separable spinel as a promising materials for photocatalysis and waste water treatment. ES Energy Environ
Malik AQ, Tahir ul Gani M, Amin O, Sathish M, Kumar D (2022b) Synthesis, characterization, photocatalytic effect of CuS-ZnO nanocomposite on photodegradation of Congo Red and phenol pollutant. Inorg Chem Commun 109797
Malik AQ, Lokhande P, Kumar D, Mooney J, Sharma A, Gani Mir TU (2023) Photocatalytic 1 and antimicrobial activity study for cadmium sulphide quantum dots. Mater Res Innov 1–9
Malik AQ, Kumar D (2023) An overview of paclitaxel and molecular imprinted polymers capped with quantum dots as an alternative approach for paclitaxel extraction and detection. Curr Mater Sci: Formerly: Recent Patents on Materials Science 16(2):185–216
CAS Google Scholar
Mallikarjunaswamy C, Lakshmi Ranganatha V, Ramu R, Udayabhanu, Nagaraju G (2020) Facile microwave-assisted green synthesis of ZnO nanoparticles: application to photodegradation, antibacterial and antioxidant. J Mater Sci: Mater Electron 31(2):1004–1021. https://doi.org/10.1007/s10854-019-02612-2
Manjunath HulikereJoshi MC (2017) Characterization, antioxidant and antimicrobial activity of silver nanoparticles synthesized using marine endophytic fungus- Cladosporium cladosporioides. Biochem Biophys Rep. https://doi.org/10.1016/j.bbrep.2017.08.011
Masoumbaigi H, Rezaee A, Hosseini H, Hashemi S (2015) Water disinfection by zinc oxide nanoparticle prepared with solution combustion method. Desalin Water Treat 56(9):2376–2381. https://doi.org/10.1080/19443994.2014.961556
Menon S, Devi S, Agarwal H, Kumar V (2019) Efficacy of biogenic selenium nanoparticles from an extract of ginger towards evaluation on anti-microbial and anti-oxidant activities. Colloid Interface Sci Commun 29:1–8. https://doi.org/10.1016/j.colcom.2018.12.004
Mir ul TG, Shukla S, Malik AQ, Singh J, Kumar D (2023) Microwave-assisted synthesis of N-doped carbon quantum dots for detection of methyl orange in saffron. Chem Pap 1–9
Mishra D, Rajurkar S, Mishra N, Jadhav N, Ballurkar B (2017) Green synthesis of gold nanoparticles by Azadirachta indica leaf extract and coating with Morinda citrifolia fruit extract: their characterization. Int J Livest Res 1. https://doi.org/10.5455/ijlr.20170306091736
Mohammadi A, Hashemi M, Hosseini SM (2015) Chitosan nanoparticles loaded with Cinnamomum zeylanicum essential oil enhance the shelf life of cucumber during cold storage. Postharvest Biol Technol 110:203–213
Mohanpuria P, Rana NK, Yadav SK (2008) Biosynthesis of nanoparticles: technological concepts and future applications. J Nanopart Res 10(3):507–517. https://doi.org/10.1007/s11051-007-9275-x
Mohanta YK, Nayak D, Biswas K, Singdevsachan SK, Abd-Allah EF, Hashem A, Alqarawi AA, Yadav D, Mohanta TK (2018) Silver nanoparticles synthesized using wild mushroom show potential antimicrobial activities against food borne pathogens. Molecules : A Journal of Synthetic Chemistry and Natural Product Chemistry 23(3):655. https://doi.org/10.3390/molecules23030655
Mohmed A, Fouda A, Abdel-Rahman M, Hassan S, Gamal S, Salah Salem S, Shaheen Th I (2019). Fungal strain impacts the shape, bioactivity and multifunctional properties of green synthesized zinc oxide nanoparticle. Biocatal Agric Biotechnol 19. https://doi.org/10.1016/j.bcab.2019.101103
Monowar T, Rahman MS, Bhore SJ, Raju G, Sathasivam KV (2018) Silver nanoparticles synthesized by using the endophytic bacterium pantoea ananatis are promising antimicrobial agents against multidrug resistant bacteria. Molecules (basel, Switzerland) 23(12):E3220. https://doi.org/10.3390/molecules23123220
Mukarram M, Khan MM, Corpas F (2021) Silicon nanoparticles elicit an increase in lemongrass (Cymbopogon flexuosus (Steud.) Wats) agronomic parameters with a higher essential oil yield. J Hazard Mater. https://doi.org/10.1016/j.jhazmat.2021.125254
Muñiz Diaz R, Cardoso-Avila PE, Pérez Tavares JA, Patakfalvi R, Villa Cruz V, Ladrón P, de Guevara H, Gutiérrez Coronado O, Arteaga Garibay RI, Saavedra Arroyo QE, Marañón-Ruiz VF, Castañeda Contreras J (2021) Two-step triethylamine-based synthesis of MgO nanoparticles and their antibacterial effect against pathogenic bacteria. Nanomaterials 11(2):410. https://doi.org/10.3390/nano11020410
Muthukumar H, Palanirajan SK, Shanmugam MK, Arivalagan P, Gummadi SN (2022) Photocatalytic degradation of caffeine and E. coli inactivation using silver oxide nanoparticles obtained by a facile green co-reduction method. Clean Technol Environ Policy 24(4):1087–1098. https://doi.org/10.1007/s10098-021-02135-7
Nagajyothi PC, Prabhakar Vattikuti SV, Devarayapalli KC, Yoo K, Shim J, Sreekanth TVM (2020) Green synthesis: photocatalytic degradation of textile dyes using metal and metal oxide nanoparticles-latest trends and advancements. Crit Rev Environ Sci Technol 50(24):2617–2723. https://doi.org/10.1080/10643389.2019.1705103
Nasrollahzadeh M, Sajjadi M, Iravani S, Varma R (2020) Green-synthesized nanocatalysts and nanomaterials for water treatment: current challenges and future perspectives. J Hazard Mater 401:123401. https://doi.org/10.1016/j.jhazmat.2020.123401
Nayantara, Kaur P (2018) Biosynthesis of nanoparticles using eco-friendly factories and their role in plant pathogenicity: a review. Biotechnol Res Innov 2(1):63–73. https://doi.org/10.1016/j.biori.2018.09.003
Ndwandwe BK, Malinga SP, Kayitesi E, Dlamini BC (2021) Advances in green synthesis of selenium nanoparticles and their application in food packaging. Int J Food Sci Technol 56:2640–2650. https://doi.org/10.1111/ijfs.14916
Onitsuka S, Hamada T, Okamura H (2018) Preparation of antimicrobial gold and silver nanoparticles from tea leaf extracts. Colloids Surfaces B: Biointerfaces, 173. https://doi.org/10.1016/j.colsurfb.2018.09.055
Ovais M, Khalil AT, Ayaz M, Ahmad I, Nethi SK, Mukherjee S (2018) Biosynthesis of Metal nanoparticles via microbial enzymes: a mechanistic approach. Int J Mol Sci 19(12):4100. https://doi.org/10.3390/ijms19124100
Pandit C, Roy A, Ghotekar S, Khusro A, Islam MN, Emran TB, Lam SE, Khandaker MU, Bradley DA (2022) Biological agents for synthesis of nanoparticles and their applications. J King Saud Univ-Sci 34(3):101869. https://doi.org/10.1016/j.jksus.2022.101869
Pantidos N, Horsfall L (2014) Biological synthesis of metallic nanoparticles by bacteria, fungi and plants. J Nanomed Nanotechnol 5. https://doi.org/10.4172/2157-7439.1000233
Parmar M, Sanyal M (2022) Extensive study on plant mediated green synthesis of metal nanoparticles and their application for degradation of cationic and anionic dyes. Environ Nanotechnol Monit Manag 17:100624. https://doi.org/10.1016/j.enmm.2021.100624
Parveen K, Banse V, Ledwani L (2016) Green synthesis of nanoparticles: their advantages and disadvantages. 1724(1):020048
Patra JK, Baek K-H (2015) Green nanobiotechnology: factors affecting synthesis and characterization techniques. J Nanomater 2014(219):219. https://doi.org/10.1155/2014/417305
Petla RK, Vivekanandhan S, Misra M, Mohanty A, Satyanarayana N (2012) Soybean (glycine max) leaf extract based green synthesis of palladium nanoparticles. J Biomater Nanobiotechnol 03. https://doi.org/10.4236/jbnb.2012.31003
Phan HT, Haes AJ (2019) What does nanoparticle stability mean? J Phys Chem C 123(27):16495–16507
Pollmann K, Raff J, Merroun M, Fahmy K, Selenska-Pobell S (2006) Metal binding by bacteria from uranium mining waste piles and its technological applications. Biotechnol Adv 24(1):58–68. https://doi.org/10.1016/j.biotechadv.2005.06.002
Prabhu P, Jose V, Lee J (2020) Heterostructured catalysts for electrocatalytic and photocatalytic carbon dioxide reduction. Adv Func Mater 30(24):1910768
Prabhu DM, Cheng J, Chen W, Sunkara A, Mane S, RamKumar DasM, Hozzein W, Duan Y-Q, Li W-J (2016) Sunlight mediated synthesis of silver nanoparticles by a novel actinobacterium (Sinomonas mesophila MPKL 26) and its antimicrobial activity against multi drug resistant Staphylococcus aureus. J Photochem Photobiol B Biol
Pugazhenthiran N, Anandan S, Kathiravan G, Udaya Prakash NK, Crawford S, Ashokkumar M (2009) Microbial synthesis of silver nanoparticles by Bacillus sp. J Nanopart Res 11(7):1811. https://doi.org/10.1007/s11051-009-9621-2
Pulit-Prociak J, Banach M (2016) Silver nanoparticles – a material of the future…? Open Chem 14(1):76–91. https://doi.org/10.1515/chem-2016-0005
Qureshi A, Blaisi NI, Abbas AAO, Khan NA, Rehman S (2021) Prospectus and development of microbes mediated synthesis of nanoparticles. In Ansari MA, Rehman S (Eds.), Microbial Nanotechnology: Green Synthesis and Applications. Springer, pp. 1–15, https://doi.org/10.1007/978-981-16-1923-6_1
Rai R, JamunaBai A (2011) Nanoparticles and their potential application as antimicrobials. Undefined. https://www.semanticscholar.org/paper/Nanoparticles-and-their-potential-application-as-Rai-JamunaBai/386a4fd085f3d53f8cdd53a4f75a4d276aaa4960
Rajabairavi N, Chellappan SR, Raju C, Karthikeyan K, Varutharaju S, Nethaji A, Hameed H, Hameed A, Shajahan, Rajabairavi N, Raju C, Karthikeyan C, Hameed Á, Varutharaju K, Shajahan A, Nethaji S (2017) Biosynthesis of novel zinc oxide nanoparticles (ZnO NPs) using endophytic bacteria Sphingobacterium thalpophilum, pp. 245–254. https://doi.org/10.1007/978-3-319-44890-9_23
Ramteke C, Chakrabarti T, Sarangi BK, Pandey R-A (2012) Synthesis of silver nanoparticles from the aqueous extract of leaves of Ocimum sanctum for enhanced antibacterial activity. J Chem 2013:e278925. https://doi.org/10.1155/2013/278925
Rasheed P, Haq S, Waseem M, Rehman S, Rehman W, Bibi N, Shah SA (2020) Green synthesis of vanadium oxide-zirconium oxide nanocomposite for the degradation of methyl orange and picloram. Mater Res Express 7. https://doi.org/10.1088/2053-1591/ab6fa2
Rashmi BN, Harlapur SF, Avinash B, Ravikumar CR, Nagaswarupa HP, Anil Kumar MR, Gurushantha K, Santosh MS (2020) Facile green synthesis of silver oxide nanoparticles and their electrochemical, photocatalytic and biological studies. Inorg Chem Commun 111:107580. https://doi.org/10.1016/j.inoche.2019.107580
Razavi R, Molaei R, Moradi M, Tajik H, Ezati P, Shafipour Yordshahi A (2020) Biosynthesis of metallic nanoparticles using mulberry fruit (Morus alba L.) extract for the preparation of antimicrobial nanocellulose film. Appl Nanosc. https://doi.org/10.1007/s13204-019-01137-8
Reischauer S, Pieber B (2021) Emerging concepts in photocatalytic organic synthesis. Iscience 24(3):102209
Restrepo CV, Villa CC (2021) Synthesis of silver nanoparticles, influence of capping agents, and dependence on size and shape: a review. Environ Nanotechnol Monit Manag 15:100428
Rice C, Ha S, Masel RI, Waszczuk P, Wieckowski A, Barnard T (2002) Direct formic acid fuel cells. J Power Sources 111(1):83–89. https://doi.org/10.1016/S0378-7753(02)00271-9
Roopan S, Annadurai B, Rajendran K, Khanna G, Arunachalam P (2012) Acaricidal, insecticidal and Larvicidal efficacy of aqueous extract of Annona squamosa peel as biomaterial for the reduction of palladium salts into nanoparticles. Colloids Surf, B 92:209–212. https://doi.org/10.1016/j.colsurfb.2011.11.044
Roy A, Elzaki A, Tirth V, Kajoak S, Osman H, Algahtani A, Islam S, Faizo NL, Khandaker MU, Islam MN, Emran TB, Bilal M (2021) Biological synthesis of nanocatalysts and their applications. Catalysts 11(12), Article 12. https://doi.org/10.3390/catal11121494
Sadegh H, Ali GAM, Gupta VK, Makhlouf ASH, Shahryari-Ghoshekandi R, Nadagouda MN, Sillanpää M, Megiel E (2017) The role of nanomaterials as effective adsorbents and their applications in wastewater treatment. J Nanostruct Chem 7(1), Article 1. https://cyberleninka.org/article/n/1479098
Safaepour M, Shahverdi AR, Shahverdi H, Khorramizadeh MR, Gohari A (2009) Green synthesis of small silver nanoparticles using geraniol and its cytotoxicity against fibrosarcoma-Wehi 164. Avicenna J Med Biotechnol 1:111–115
Salem TA, Fetian NA, Elsheery NI (2019) Nanotechnology for Polluted soil remediation. In Panpatte DG, Jhala YK (Eds.), Nanotechnology for Agriculture: Advances for Sustainable Agriculture. Springer, pp. 285–305, https://doi.org/10.1007/978-981-32-9370-0_15
Salem SS, Fouda A (2021) Green synthesis of metallic nanoparticles and their prospective biotechnological applications: an overview. Biol Trace Elem Res 199:344–370
Salimi M, Rassi Y, Chatrabgoun O, Kamali A, Oshaghi MA, Shiri-Ghaleh V, Moradi M, Rafizadeh S, Akbarzadeh K, Parkhideh SZ (2018) Toxicological analysis of insects on the corpse: a valuable source of information in forensic investigations. J Arthropod-Borne Dis 12(3):219–231. https://doi.org/10.18502/jad.v12i3.74 . (Scopus)
Sangaru SS, Rai A, Ahmad A, Sastry M (2004) Biosynthesis of silver and gold nanoparticles from extracts of different parts of the geranium plant. Appl Nanosci 1:69–77
Google Scholar
Santhosh C, Velmurugan V, Jacob G, Jeong SK, Grace AN, Bhatnagar A (2016) Role of nanomaterials in water treatment applications: a review. Chem Eng J 306:1116–1137. https://doi.org/10.1016/j.cej.2016.08.053
Saratale RG, Saratale GD, Shin HS, Jacob JM, Pugazhendhi A, Bhaisare M, Kumar G (2018) New insights on the green synthesis of metallic nanoparticles using plant and waste biomaterials: current knowledge, their agricultural and environmental applications. Environ Sci Pollut Res 25:10164–10183
Sarathy V, Tratnyek PG, Nurmi JT, Baer DR, Amonette JE, Chun CL, Penn RL, Reardon EJ (2008) Aging of iron nanoparticles in aqueous solution: effects on structure and reactivity. J Phys Chem C 112(7):2286–2293. https://doi.org/10.1021/jp0777418
Saravanakumar K, Hu X, Chelliah R, Oh D-H, Kathiresan K, Wang M-H (2020) Biogenic silver nanoparticles-polyvinylpyrrolidone based glycerosomes coating to expand the shelf life of fresh-cut bell pepper (Capsicum annuum L. var. Grossum (L.) Sendt). Postharvest Biol Technol 160:111039
Saravanan M, Barik SK, MubarakAli D, Prakash P, Pugazhendhi A (2018) Synthesis of silver nanoparticles from Bacillus brevis (NCIM 2533) and their antibacterial activity against pathogenic bacteria. Microb Pathog 116:221–226. https://doi.org/10.1016/j.micpath.2018.01.038
Schlüter M, Hentzel T, Suarez C, Koch M, Lorenz W, Böhm L, Duering R-A, Koinig K, Bunge M (2014) Synthesis of novel palladium(0) nanocatalysts by microorganisms from heavy-metal-influenced high-alpine sites for dehalogenation of polychlorinated dioxins. Chemosphere 117C:462–470. https://doi.org/10.1016/j.chemosphere.2014.07.030
Schubert J, Chanana M (2019) Coating matters: Review on colloidal stability of nanoparticles with biocompatible coatings in biological media, living cells and organisms. Curr Med Chem 25(35):4556
Seifunnisha O, Jayaraj S (2020) Aloe vera mediated green synthesis of ZnO nanostructure under sol-gel method: effect of antimicrobial activity. J Nano-Electron Phys 12:02041–1. https://doi.org/10.21272/jnep.12(2).02041
Seil JT, Webster TJ (2012) Antimicrobial applications of nanotechnology: methods and literature. Int J Nanomed 7:2767–2781. https://doi.org/10.2147/IJN.S24805
Shahzad A, Iqtedar M, Saeed H, Hussain SZ, Chaudhary A, Abdullah R, Kaleem A (2019) Mycosynthesis of size-controlled silver nanoparticles through optimization of process variables by response surface methodology. Polish J Microbiol 68(1):35–42. https://doi.org/10.21307/pjm-2019-004
Shamprasad BR, Lotha R, Nagarajan S, Sivasubramanian A (2022) Metal nanoparticles functionalized with nutraceutical Kaempferitrin from edible Crotalaria juncea, exert potent antimicrobial and antibiofilm effects against Methicillin-resistant Staphylococcus aureus. Sci Rep 12. https://doi.org/10.1038/s41598-022-11004-2
Shehab MM, Elbialy ZI, Tayel AA, Moussa SH, Al-Hawary II (2022) Quality boost and shelf-life prolongation of african catfish fillet using Lepidium sativum mucilage extract and selenium nanoparticles. J Food Qual 2022
Shipley HJ, Engates KE, Guettner AM (2011) Study of iron oxide nanoparticles in soil for remediation of arsenic. J Nanopart Res 13(6):2387–2397. https://doi.org/10.1007/s11051-010-9999-x
Shubha JP, Kavalli K, Adil SF, Assal ME, Hatshan MR, Dubasi N (2022) Facile green synthesis of semiconductive ZnO nanoparticles for photocatalytic degradation of dyes from the textile industry: a kinetic approach. J King Saud Univ-Sci 34(5):102047. https://doi.org/10.1016/j.jksus.2022.102047
Singh M, Kalaivani R, Manikandan S, Sangeetha N, Kumaraguru AK (2013) Facile green synthesis of variable metallic gold nanoparticle using Padina gymnospora, a brown marine macroalga. Appl Nanosci 3(2):145–151. https://doi.org/10.1007/s13204-012-0115-7
Singh K, Panghal M, Kadyan S, Chaudhary U, Yadav JP (2014) Green silver nanoparticles of Phyllanthus amarus: as an antibacterial agent against multi drug resistant clinical isolates of Pseudomonas aeruginosa. J Nanobiotechnol 12:40. https://doi.org/10.1186/s12951-014-0040-x
Singh J, Dutta T, Kim K-H, Rawat M, Samddar P, Kumar P (2018) ‘Green’ synthesis of metals and their oxide nanoparticles: applications for environmental remediation. J Nanobiotechnol 16(1):84. https://doi.org/10.1186/s12951-018-0408-4
Sintubin L, De Windt W, Dick J, Mast J, van der Ha D, Verstraete W, Boon N (2009) Lactic acid bacteria as reducing and capping agent for the fast and efficient production of silver nanoparticles. Appl Microbiol Biotechnol 84(4):741–749. https://doi.org/10.1007/s00253-009-2032-6
Siripireddy B, Mandal BK (2017) Facile green synthesis of zinc oxide nanoparticles by Eucalyptus globulus and their photocatalytic and antioxidant activity. Adv Powder Technol 28(3):785–797. https://doi.org/10.1016/j.apt.2016.11.026
Song Y, Chen L (2015) Effect of net surface charge on physical properties of the cellulose nanoparticles and their efficacy for oral protein delivery. Carbohyd Polym 121:10–17. https://doi.org/10.1016/j.carbpol.2014.12.019
Soto-Robles CA, Luque PA, Gómez-Gutiérrez CM, Nava O, Vilchis-Nestor AR, Lugo-Medina E, Ranjithkumar R, Castro-Beltrán A (2019) Study on the effect of the concentration of Hibiscus sabdariffa extract on the green synthesis of ZnO nanoparticles. Results Phys 15:102807. https://doi.org/10.1016/j.rinp.2019.102807
Soundarrajan C, Sankari A, Dhandapani P, Maruthamuthu S, Ravichandran S, Sozhan G, Palaniswamy N (2011) Rapid biological synthesis of platinum nanoparticles using Ocimum sanctum for water electrolysis applications. Bioprocess Biosyst Eng 35:827–833. https://doi.org/10.1007/s00449-011-0666-0
Sreekanth TVM, Nagajyothi PC, Muthuraman P, Enkhtaivan G, Vattikuti SVP, Tettey CO, Kim DH, Shim J, Yoo K (2018) Ultra-sonication-assisted silver nanoparticles using Panax ginseng root extract and their anti-cancer and antiviral activities. J Photochem Photobiol, B 188:6–11. https://doi.org/10.1016/j.jphotobiol.2018.08.013
Srinath BS, Namratha K, Byrappa K (2018) Eco-friendly synthesis of gold nanoparticles by Bacillus subtilis and their environmental applications. Adv Sci Lett 24(8):5942–5946. https://doi.org/10.1166/asl.2018.12224
Subba Rao Y, Kotakadi VS, Prasad TNVKV, Reddy AV, Sai Gopal DVR (2013) Green synthesis and spectral characterization of silver nanoparticles from Lakshmi tulasi (Ocimum sanctum) leaf extract. Spectrochim Acta Part A Mol Biomol Spectrosc 103:156–159. https://doi.org/10.1016/j.saa.2012.11.028
Subhapriya S, Gomathipriya P (2018) Green synthesis of titanium dioxide (TiO2) nanoparticles by Trigonella foenum-graecum extract and its antimicrobial properties. Microb Pathog 116:215–220. https://doi.org/10.1016/j.micpath.2018.01.027
Sumi MB, Devadiga A, Vidya Shetty K, Saidutta MB (2017) Solar photocatalytically active, engineered silver nanoparticle synthesis using aqueous extract of mesocarp of Cocos nucifera (Red Spicata Dwarf). J Exp Nanosci 12(1):14–32. https://doi.org/10.1080/17458080.2016.1251622
Suriyaprom S, Kaewkod T, Promputtha I, Desvaux M, Tragoolpua Y (2021) Evaluation of antioxidant and antibacterial activities of white mulberry (Morus alba L.) fruit extracts. Plants 10(12):2736. https://doi.org/10.3390/plants10122736
Suryavanshi P, Pandit R, Gade A, Derita M, Zachino S, Rai M (2017) Colletotrichum sp.- mediated synthesis of sulphur and aluminium oxide nanoparticles and its in vitro activity against selected food-borne pathogens. LWT - Food Sci Technol 81. https://doi.org/10.1016/j.lwt.2017.03.038
Tagad CK, Dugasani SR, Aiyer R, Park S, Kulkarni A, Sabharwal S (2013) Green synthesis of silver nanoparticles and their application for the development of optical fiber based hydrogen peroxide sensor. Sens Actuators, B Chem 183:144–149
Tahir K, Nazir S, Ahmad A, Li B, Khan AU, Khan ZUH, Khan F, Khan Q, Khan A, Rehman A (2016) Facile and green synthesis of phytochemicals capped platinum nanoparticles and in vitro their superior antibacterial activity. J Photochem Photobiol B, Biology 166:246–251. https://doi.org/10.1016/j.jphotobiol.2016.12.016
Tahir M, Sagir M, Abas N (2019) Enhanced photocatalytic performance of CdO-WO3 composite for hydrogen production. Int J Hydrogen Energy. https://doi.org/10.1016/j.ijhydene.2019.07.220
Taran M, Rad M, Alavi M (2018) Biosynthesis of TiO2 and ZnO nanoparticles by Halomonas elongata IBRC-M 10214 in different conditions of medium. BioImpacts: BI 8(2):81–89. https://doi.org/10.15171/bi.2018.10
Thangavelu RM, Ganapathy R, Ramasamy P, Krishnan K (2020) Fabrication of virus metal hybrid nanomaterials: an ideal reference for bio semiconductor. Arab J Chem 13(1):2750–2765. https://doi.org/10.1016/j.arabjc.2018.07.006
Thomas R, Janardhanan A, Varghese RT, Soniya EV, Mathew J, Radhakrishnan EK (2014) Antibacterial properties of silver nanoparticles synthesized by marine Ochrobactrum sp. Braz J Microbiol: [publication of the Brazilian Society for Microbiology] 45(4):1221–1227. https://doi.org/10.1590/s1517-83822014000400012
Tiu BDB, Kernan DL, Tiu SB, Wen AM, Zheng Y, Pokorski JK, Advincula RC, Steinmetz NF (2017) Electrostatic layer-by-layer construction of fibrous TMV biofilms. Nanoscale 9(4):1580–1590. https://doi.org/10.1039/c6nr06266k
Tripathi A, Liu S, Singh PK, Kumar N, Pandey AC, Tripathi DK, Chauhan DK, Sahi S (2017) Differential phytotoxic responses of silver nitrate (AgNO3) and silver nanoparticle (AgNps) in Cucumis sativus L. Plant Gene. https://doi.org/10.1016/j.plgene.2017.07.005
Tungittiplakorn W, Lion LW, Cohen C, Kim J-Y (2004) Engineered polymeric nanoparticles for soil remediation. Environ Sci Technol 38(5):1605–1610. https://doi.org/10.1021/es0348997
ul Gani Mir T, Malik AQ, Singh J, Shukla S, Kumar D (2022) An overview of molecularly imprinted polymers embedded with quantum dots and their implementation as an alternative approach for extraction and detection of Crocin. ChemistrySelect 7(21):e202200829
Valodkar M, Jadeja RN, Thounaojam MC, et al (2011) Biocompatible synthesis of peptide capped copper nanoparticles and their biological effect on tumor cells. Mater Chem Phys 128:83–89. https://doi.org/10.1016/j.matchemphys.2011.02.039
Velayutham K, Rahuman AA, Rajakumar G, Santhoshkumar T, Marimuthu S, Jayaseelan C, Bagavan A, Kirthi AV, Kamaraj C, Zahir AA, Elango G (2012) Evaluation of Catharanthus roseus leaf extract-mediated biosynthesis of titanium dioxide nanoparticles against Hippobosca maculata and Bovicola ovis. Parasitol Res 111(6):2329–2337. https://doi.org/10.1007/s00436-011-2676-x
Waglewska E, Pucek-Kaczmarek A, Bazylińska U (2020) Novel surface-modified bilosomes as functional and biocompatible nanocarriers of hybrid compounds. Nanomaterials 10(12):2472
Wang W, Xu X, Zhou W, Shao Z (2017) Recent progress in metal-organic frameworks for applications in electrocatalytic and photocatalytic water splitting. Adv Sci 4(4):1600371
Wang Y, O’Connor D, Shen Z, Lo IMC, Tsang DCW, Pehkonen S, Pu S, Hou D (2019) Green synthesis of nanoparticles for the remediation of contaminated waters and soils: constituents, synthesizing methods, and influencing factors. J Clean Prod 226:540–549. https://doi.org/10.1016/j.jclepro.2019.04.128
Wang P, Wang F, Jiang H, Zhang Y, Zhao M, Xiong R, Ma J (2020) Strong improvement of nanofiltration performance on micropollutant removal and reduction of membrane fouling by hydrolyzed-aluminum nanoparticles. Water Res 175:115649. https://doi.org/10.1016/j.watres.2020.115649
Wang W-N, Tarafdar J, Biswas P (2013) Nanoparticle synthesis and delivery by an aerosol route for watermelon plant foliar uptake. J Nanopart Res 15. https://doi.org/10.1007/s11051-013-1417-8
Wani AK, Akhtar N, Mir ul TG, Singh R, Jha PK, Mallik SK, Sinha S, Tripathi SK, Jain A, Jha A (2023) Targeting apoptotic pathway of cancer cells with phytochemicals and plant-based nanomaterials. Biomolecules 13(2):194
Wypij M, Czarnecka J, Świecimska M, Dahm H, Rai M, Golinska P (2018) Synthesis, characterization and evaluation of antimicrobial and cytotoxic activities of biogenic silver nanoparticles synthesized from Streptomyces xinghaiensis OF1 strain. World J Microbiol Biotechnol 34(2):23. https://doi.org/10.1007/s11274-017-2406-3
Xiao Q, Jaatinen E, Zhu H (2014) Direct photocatalysis for organic synthesis by using plasmonic-metal nanoparticles irradiated with visible light. Chem–An Asian J 9(11):3046–3064
Xu J, Huang Y, Zhu S, Abbes N, Jing X, Zhang L (2021) A review of the green synthesis of ZnO nanoparticles using plant extracts and their prospects for application in antibacterial textiles. J Eng Fibers Fabr 16:15589250211046242. https://doi.org/10.1177/15589250211046242
Yurekli Y (2016) Removal of heavy metals in wastewater by using zeolite nano-particles impregnated polysulfone membranes. J Hazard Mater 309:53–64. https://doi.org/10.1016/j.jhazmat.2016.01.064
Zhang W, Xiao B, Fang T (2018a) Chemical transformation of silver nanoparticles in aquatic environments: mechanism, morphology and toxicity. Chemosphere 191:324–334
Zhang Y, Dong Y, Zhou J, Li X, Wang F (2018b) Application of plant viruses as a biotemplate for nanomaterial fabrication. Molecules 23(9), Article 9. https://doi.org/10.3390/molecules23092311
Zhang D, Ma X, Gu Y, Huang H, Zhang G (2020) Green Synthesis of metallic nanoparticles and their potential applications to treat cancer. Front Chem 8. https://www.frontiersin.org/articles/10.3389/fchem.2020.00799
Zhao C, Wang B, Theng BKG, Wu P, Liu F, Wang S, Lee X, Chen M, Li L, Zhang X (2021) Formation and mechanisms of nano-metal oxide-biochar composites for pollutants removal: a review. Sci Total Environ 767:145305. https://doi.org/10.1016/j.scitotenv.2021.145305
Download references
Author information
Authors and affiliations.
School of Bioengineering and Biosciences, Lovely Professional University, Phagwara, Punjab, India, 144411
Azad Qayoom Malik, Deepak Kumar, Irtiqa Ashraf Mir, Adfar Rashid & Mehnaz Ayoub
School of Chemical Engineering and Physical Sciences, Lovely Professional University, Phagwara, Punjab, India, 144411
Tahir ul Gani Mir & Saurabh Shukla
You can also search for this author in PubMed Google Scholar
Contributions
Conceptualization: Azad Qayoom Malik, Tahir ul Gani Mir; data collection: Azad Qayoom Malik, Adfar Rashid, Mehnaz Ayoub, Irtiqa Ashraf Mir; draft manuscript preparation: Tahir ul Gani Mir, Azad Qayoom Malik; review and editing: Deepak Kumar, Saurabh Shukla; supervision/visualization: Deepak Kumar, Saurabh Shukla.
Corresponding author
Correspondence to Azad Qayoom Malik .
Ethics declarations
Ethical approval, consent to participate, consent for publication.
All authors reviewed and approved the final version of the manuscript.
Conflict of interest
The authors declare no competing interests.
Additional information
Responsible Editor: George Z. Kyzas
Publisher's note
Springer Nature remains neutral with regard to jurisdictional claims in published maps and institutional affiliations.
Rights and permissions
Springer Nature or its licensor (e.g. a society or other partner) holds exclusive rights to this article under a publishing agreement with the author(s) or other rightsholder(s); author self-archiving of the accepted manuscript version of this article is solely governed by the terms of such publishing agreement and applicable law.
Reprints and permissions
About this article
Malik, A.Q., Mir, T.u.G., Kumar, D. et al. A review on the green synthesis of nanoparticles, their biological applications, and photocatalytic efficiency against environmental toxins. Environ Sci Pollut Res 30 , 69796–69823 (2023). https://doi.org/10.1007/s11356-023-27437-9
Download citation
Received : 04 March 2023
Accepted : 01 May 2023
Published : 12 May 2023
Issue Date : June 2023
DOI : https://doi.org/10.1007/s11356-023-27437-9
Share this article
Anyone you share the following link with will be able to read this content:
Sorry, a shareable link is not currently available for this article.
Provided by the Springer Nature SharedIt content-sharing initiative
- Green synthesis
- Nanoparticless
- Environment remediation
- Water treatment
- Photocatalysis
- Antimicrobial activity
- Find a journal
- Publish with us
- Track your research
- My Shodhganga
- Receive email updates
- Edit Profile
Shodhganga : a reservoir of Indian theses @ INFLIBNET
- Shodhganga@INFLIBNET
- Hemwati Nandan Bahuguna Garhwal University
- Department of English
Items in Shodhganga are licensed under Creative Commons Licence Attribution-NonCommercial-ShareAlike 4.0 International (CC BY-NC-SA 4.0).

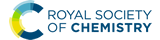
Nanomaterials: a review of synthesis methods, properties, recent progress, and challenges

First published on 24th February 2021
Nanomaterials have emerged as an amazing class of materials that consists of a broad spectrum of examples with at least one dimension in the range of 1 to 100 nm. Exceptionally high surface areas can be achieved through the rational design of nanomaterials. Nanomaterials can be produced with outstanding magnetic, electrical, optical, mechanical, and catalytic properties that are substantially different from their bulk counterparts. The nanomaterial properties can be tuned as desired via precisely controlling the size, shape, synthesis conditions, and appropriate functionalization. This review discusses a brief history of nanomaterials and their use throughout history to trigger advances in nanotechnology development. In particular, we describe and define various terms relating to nanomaterials. Various nanomaterial synthesis methods, including top-down and bottom-up approaches, are discussed. The unique features of nanomaterials are highlighted throughout the review. This review describes advances in nanomaterials, specifically fullerenes, carbon nanotubes, graphene, carbon quantum dots, nanodiamonds, carbon nanohorns, nanoporous materials, core–shell nanoparticles, silicene, antimonene, MXenes, 2D MOF nanosheets, boron nitride nanosheets, layered double hydroxides, and metal-based nanomaterials. Finally, we conclude by discussing challenges and future perspectives relating to nanomaterials.
1. Introduction
The term nanometer was first used in 1914 by Richard Adolf Zsigmondy. 5 The American physicist and Nobel Prize laureate Richard Feynman introduced the specific concept of nanotechnology in 1959 in his speech during the American Physical Society's annual meeting. This is considered to be the first academic talk about nanotechnology. 5 He presented a lecture that was entitled “There's Plenty of Room at the Bottom”. During this meeting, the following concept was presented: “why can’t we write the entire 24 volumes of the Encyclopedia Britannica on the head of a pin?” The vision was to develop smaller machines, down to the molecular level. 6,7 In this talk, Feynman explained that the laws of nature do not limit our ability to work at the atomic and molecular levels, but rather it is a lack of appropriate equipment and techniques that limit this. 8 Through this, the concept of modern technology was seeded. Due to this, he is often considered the father of modern nanotechnology. Norio Taniguchi might be the first person who used the term nanotechnology, in 1974. Norio Taniguchi stated: “nano-technology mainly consists of the processing of, separation, consolidation, and deformation of materials by one atom or one molecule.” 5,9 Before the 1980s, nanotechnology remained only an area for discussion, but the concept of nanotechnology was seeded in the minds of researchers with the potential for future development.
The invention of various spectroscopic techniques sped up research and innovations in the field of nanotechnology. IBM researchers developed scanning tunneling microscopy (STM) in 1982, and with STM it became feasible to attain images of single atoms on “flat” ( i.e. , not a tip) surfaces. 10 Atomic force microscopy (AFM) was invented in 1986, and it has become the most crucial scanning probe microscope technique. 11 The motivation to develop hard discs with high storage density stimulated the measurement of electrostatic and magnetic forces. This led to the development of Kelvin-probe-, electrostatic-, and magnetic-force microscopy. 12 Currently, nanotechnology is rapidly evolving and becoming part of almost every field related to materials chemistry. The field of nanotechnology is evolving every day, and now powerful characterization and synthesis tools are available for producing nanomaterials with better-controlled dimensions.
Nanotechnology is an excellent example of an emerging technology, offering engineered nanomaterials with the great potential for producing products with substantially improved performances. 13 Currently, nanomaterials find commercial roles in scratch-free paints, surface coatings, electronics, cosmetics, environmental remediation, sports equipment, sensors, and energy-storage devices. 14 This review attempts to provide information in a single platform about the basic concepts, advances, and trends relating to nanomaterials via covering the related information and discussing synthesis methods, properties, and possible opportunities relating to the broad and fascinating area of nanomaterials ( Scheme 1 ). It is not easy to cover all the literature related to nanomaterials, but important papers from history and the current literature are discussed in this review. This review provides fundamental insight for researchers, quickly capturing the advances in and properties of various classes of nanomaterials in one place.
2. Descriptions of terms associated with nanomaterials
3. approaches for the synthesis of nanomaterials, 3.1. top-down approaches.
The conditions under which arc discharge takes place play a significant role in achieving new forms of nanomaterials. The conditions under which different carbon-based nanomaterials are formed via the arc discharge method are explained in Fig. 6 . Various carbon-based nanomaterials are collected from different positions during the arc discharge method, as their growth mechanisms differ. 44 MWCNTs, high-purity polyhedral graphite particles, pyrolytic graphite, and nano-graphite particles can be collected from either anode or cathode deposits or deposits at both electrodes. 46–48 Apart from the electrodes, carbon-based nanomaterials can also be collected from the inner chamber. Different morphologies of single-wall carbon nanohorns (SWCNHs) can be obtained under different atmospheres. For example, ‘dahlia-like’ SWCNHs are produced under an ambient atmosphere, whereas ‘bud-like’ SWCNHs are generated under CO and CO 2 atmospheres. 49 The arc discharge method can be used to efficiently achieve graphene nanostructures. The conditions present during the synthesis of graphene can affect its properties. Graphene sheets prepared via a hydrogen arc discharge exfoliation method are found to be superior in terms of electrical conductivity and have good thermal stability compared to those obtained via argon arc discharge. 50
3.2. Bottom-up approaches
The hard template method is also called nano-casting. Well-designed solid materials are used as templates, and the solid template pores are filled with precursor molecules to achieve nanostructures for required applications ( Fig. 10 ). 78 The selection of the hard template is critical for developing well-ordered mesoporous materials. It is desirable that such hard templates should maintain a mesoporous structure during the precursor conversion process, and they should be easily removable without disrupting the produced nanostructure. A range of materials has been used as hard templates, not limited to carbon black, silica, carbon nanotubes, particles, colloidal crystals, and wood shells. 85 Three main steps are involved in the synthetic pathway for obtaining nanostructures via templating methods. In the first step, the appropriate original template is developed or selected. Then, a targeted precursor is filled into the template mesopores to convert them into an inorganic solid. In the final step, the original template is removed to achieve the mesoporous replica. 86 Via using mesoporous templates, unique nanostructured materials such as nanowires, nanorods, 3D nanostructured materials, nanostructured metal oxides, and many other nanoparticles can be produced. 87 From this brief discussion, it can be seen that a wide range of unique structured nanomaterials can be produced using soft and hard template methods.
4. Unique nanomaterial features
The electronic properties of semiconductors in the 1–10 nm range are controlled by quantum mechanical considerations. Thus, nanospheres with diameters in the range of 1–10 nm are known as quantum dots. The optical properties of nanomaterials such as quantum dots strongly depend upon their shape and size. 96 A photogenerated electron–hole pair has an exciton diameter on the scale of 1–10 nm. Thus, the absorption and emission of light by semiconductors could be controlled via tuning the nanoparticle size in this range. However, in the case of metals, the mean free path of electrons is ∼10–100 nm and, due to this, electronic and optical effects are expected to be observed in the range of ∼10–100 nm. The colors of aqueous solutions of metal nanoparticles can be changed via changing the aspect ratio. Aqueous solutions of Ag NPs show different colors at different aspect ratios. A red shift in the absorption band appears with an increase in the aspect ratio ( Fig. 12 ). 21
Among a range of unique properties, the following key properties can be obtained upon tuning the sizes and morphologies of nanomaterials.
4.1. Surface area
4.2. magnetism, 4.3. quantum effects, 4.4. high thermal and electrical conductivity, 4.5. excellent mechanical properties, 4.6. excellent support for catalysts, 4.7. antimicrobial activity.
Overall, these features have made nanoscale materials valuable for a wide range of applications, substantially boosting the performances of various devices and materials in a number of fields. Details of various nanomaterials, their properties, and applications in various fields will be discussed below.
5. Nanomaterials, characteristics, and applications
5.1. special carbon-based nanomaterials.
In the carbon-based nanomaterial family, fullerenes were the first symmetric material, and they provided new perspectives in the nanomaterials field. This led to the discovery of other carbon-based nanostructured materials, such as carbon nanotubes and graphene. 110 Fullerenes are present in nature and interstellar space. 111 Interestingly, fullerenes were the molecule of the year in 1991 and attracted the most research projects compared to other scientific subjects during that period. 112 Fullerenes possess several unique features that make them attractive for applications in different fields. Fullerenes display solubility to some extent in a range of solvents, and these characteristics make them unique compared to the other allotropes of carbon. 108
The chemical modification of fullerenes is an exciting subject, improving their effectiveness for applications. There are two main ways to modify fullerenes: 113 fullerene inner-space modification, and fullerene outer-surface modification.
Endohedral and exohedral doping examples are shown in Fig. 13 . 114 Fullerenes are hollow cages, and the interior acts as a robust nano-container for host target species when forming endohedral fullerene. 115 Endohedral fullerenes do not always follow the isolated pentagon rule (IPR). 116 To date, fullerene nanocages have received substantial consideration in the materials chemistry field due to their useful potential applications. Neutral and charged single atoms in free space are highly reactive and unstable. In the confined environment of fullerenes, these reactive species can be stabilized; for example, the LaC 60 + ion does not react with the NH 3 , O 2 , H 2 , or NO. Thus, reactive metals can be protected from the surrounding environment by trapping them inside fullerene cages. 117 Another emerging carbon nanomaterial is endohedral fullerene containing lithium (Li@C 60 ). 118 Lithium metal is very reactive, and extreme controlled environmental conditions are required to preserve or use it. In other words, secure structures are required for lithium storage. Li-Based endohedral fullerene shows unique solid-state properties. The encapsulation of lithium atoms in fullerene helps to protect lithium atoms from external agents. Li-Based endohedral fullerenes have the potential to open the door to nanoscale lithium batteries. 119 For the development of endohedral metallofullerenes, larger fullerenes are generally required, as they possess large cages to accommodate lanthanide and transition metal atoms more smoothly. 118 Fullerene nanocages are useful for the storage of gases. Fullerene is under consideration for hydrogen storage. 120,121
Exohedral fullerenes carry more potential for applications as outer surfaces can be more easily modified or functionalized. The exohedral doping of metals into fullerenes strongly affects the electronic properties via shifting electrons from the metal to the fullerene nanocage. 122 The practical application of fullerenes can be achieved with tailor-made fullerene derivatives via chemical functionalization. As fullerene chemistry has matured, a wide range of functionalized fullerenes has been realized through simple synthetic routes. 123 The combination of hydrogen-bonding motifs and fullerenes has allowed the modulation of 1D, 2D, and 3D fullerene-based architectures. 124 The excellent electron affinities of fullerenes have shown great potential for eliminating reactive oxygen species. The presence of excess reactive oxygen species can cause biological dysfunction or other health issues. The surfaces of fullerenes have been functionalized via mussel-inspired chemistry and Michael addition reactions for the fabrication of C 60 –PDA–GSH. The developed C 60 –PDA–GSH nanoparticles demonstrated excellent potential for scavenging reactive oxygen species. 125
Amphiphiles have great importance in industrial processes and daily life applications. Amphiphilic molecules consist of hydrophilic and hydrophobic parts, and they perform functions in water via forming two- and three-dimensional assemblies. Recently, conical fullerene amphiphiles 126 have emerged as a new class of amphiphiles, in which a nonpolar apex is supplied by fullerenes and a hydrophilic part is achieved through functionalization. The selective functionalization of the fullerene on one side helps to achieve a supramolecule due to unique interfacial behavior. The unique supramolecular structure formed via the spontaneous assembly of one-sided selectively functionalized fullerenes through strong hydrophobic interactions between the fullerene apexes and polar functionalized portions is soluble in water. Conical fullerene amphiphiles are mechanically robust. Via upholding the structural integrity, conical fullerene amphiphiles can be readily aggregated with nanomaterials and biomolecules to form multicomponent agglomerates with controllable structural features. 127 Fullerenes, after suitable surface modification, have excellent potential for use in drug delivery, but there have only been limited explorations of their drug delivery applications. 128,129 Fullerene-based nano-vesicles have been developed for the delayed release of drugs. 130 Water-soluble proteins have great potential in the field of nanomedicine. The water-soluble cationic fullerene, tetra(piperazino)[60] fullerene epoxide (TPFE), has been used for the targeted delivery of DNA and siRNA specifically to the lungs. 131 For diseases in lungs or any other organ, efficient treatment requires the targeted delivery of active agents to a targeted place in the organ. The accumulation of micrometer-sized carriers in the lung makes lung-selective delivery difficult, as this may induce embolization and inflammation in the lungs. Size-controlled blood vessel carrier vehicles have been developed using tetra(piperazino)fullerene epoxide (TPFE). TPFE and siRNA agglutinate in the bloodstream with plasma proteins and, as a result, micrometer-sized particles are formed. These particles clog the lung capillaries and release siRNA into lungs cells; after siRNA delivery, they are immediately cleared from the lungs ( Fig. 14 ). 132
The supramolecular organization of fullerene (C 60 ) is a unique approach for producing shape-controlled moieties on the nano-, micro-, and macro-scale. Nano-, micro-, and macro-scale supramolecular assemblies can be controlled via manipulating the preparation conditions to achieve unique optoelectronic properties. 133 The development of well-ordered and organized 1D, 2D, and 3D fullerene assemblies is essential for achieving advanced optical and organic-based electronic devices. 134 Fullerene-based nanostructured materials with new dimensions are being developed from zero-dimensional fullerene and tuned to achieve the desired characteristics. 1D C 60 fullerene nanowires have received substantial attention over other crystalline forms due to their excellent features of potential quantum confinement effects, low dimensionality, and large surface areas. 135
Carbon nanomaterials are also used as supports for catalysts, and the main reasons to use them are their high surface areas and electrical conductivities. Carbon supports strongly influence the properties of metal nanoparticles. In fuel cells, the carbon support strongly affects the stability, electronic conductivity, mass transport properties, and electroactive surface area of the supported catalyst. 136 In fuel cells, the degradation of some catalysts, such as platinum-based examples, and carbon is correlated and reinforced as a result of both being present. Carbon support oxidation is catalyzed by platinum and the oxidation of carbon accelerates platinum-catalyst release. Overall, this results in a loss of catalytically active surface area. 137 Fullerenes are considered suitable support materials due to their excellent electrochemical activities and stability during electrochemical reactions. 138 Due to their high stability and good conductivity, fullerenes can replace conventional carbon as catalyst support materials. Fullerenes are also used for the development of efficient solar cells. 139
Apart from the applications mentioned above, fullerenes have a broader spectrum of applications where they can be used to improve outcomes considerably. Fullerenes have the potential to be used in the development of superconductors. 140 The strong covalent bonds in fullerenes make them useful nanomaterials for improving the mechanical properties of composites. 141 The combination of fullerenes with polymers can result in good flame-retardant and thermal properties. 142 Fullerenes and their derivatives are used for the development of advanced lubricants. They are used as modifiers for greases and individual solid lubricants. 138 Fullerenes have tremendous medicinal importance due to their anticancer, antioxidant, anti-bacterial, and anti-viral activities. 104
Fullerenes are vital members of the carbon-based nanomaterial family and they certainly possess exceptional properties. This discussion further emphasizes their importance for advanced applications. However, the discovery of other carbon-based nanomaterials has put fullerenes in the shade, and the pace of their exploration has been reduced. As fullerenes are highly symmetrical molecules with unique properties, they can act as performance boosters, but more attention is needed from researchers for their practical expansion. 110
Single-walled carbon nanotubes consist of a seamless one-atom-thick graphitic layer, in which carbon atoms are connected through strong covalent bonds. 146 Double-walled carbon nanotubes consist of two single-walled carbon nanotubes. One carbon nanotube is nested in another nanotube to construct a double-walled carbon nanotube. 147 In multi-walled carbon nanotubes, multiple sheets of single-layer carbon atom are rolled up. In other words, many single-walled carbon nanotubes are nested within each other. From different types of nanotubes, it can be concluded that the nanotubes may consist of one, tens, or hundreds of concentric carbon shells, and these shells are separated from each other with a distance of ∼0.34 nm. 148 Carbon nanotubes can be synthesized via chemical vapor deposition, 149 laser ablation, 150 arc-discharge, 143 and gas-phase catalytic growth. 151
Single-walled carbon nanotubes display a diameter of 0.4 to 2 nm. The inner wall distance between double-walled carbon nanotubes was found to be in the range of 0.33 to 0.42 nm. MWCNT diameters are usually in the range of 2–100 nm, and the inner wall distance is about 0.34 nm. 147,152 However, it is essential to note that the diameters and lengths of carbon nanotubes are not well defined, and they depend on the synthesis route and many other factors. The electrical conductivities of SWCNTs and MWCNTs are about 10 2 –10 6 S cm −1 and 10 3 –10 5 S cm −1 , respectively. SWCNTs and MWCNTs also display excellent thermal conductivities of ∼6000 W m −1 K −1 and ∼2000 W m −1 K −1 , respectively. CNTs remain stable in air at temperatures higher than 600 °C. 153 These properties indicate that CNTs have obvious advantages over graphite.
Single-walled carbon nanotubes can display metallic or semiconducting behavior. Whether carbon nanotubes show metallic or semiconducting behavior depends on the diameter and helicity of the graphitic rings. 154 The rolling of graphene sheets leads to three different types of CNTs: chiral, armchair, and zigzag ( Fig. 15 ). 155
Carbon nanotubes demonstrate some amazing characteristics that make them valuable nanomaterials for possible practical applications. Theoretical and experimental studies of carbon nanotubes have revealed their extraordinary tensile properties. J. R. Xiao et al. used an analytical molecular structural mechanics model to predict SWCNT tensile strengths of 94.5 (zigzag nanotubes) and 126.2 (armchair nanotubes) GPa. 156 In another study, the Young's modulus and average tensile strength of millimeter-long multi-walled carbon nanotubes were analyzed and found to be 34.65 GPa and 0.85 GPa, respectively. 157 Carbon nanotubes possess a high aspect ratio. Due to their high tensile strength, carbon nanotubes are used to enhance the mechanical properties of composites.
Carbon nanotubes have become an important industrial material and hundreds of tonnes are produced for applications. 158 Their high tensile strength and high aspect ratio have made carbon nanotubes an ideal reinforcing agent. 159 Carbon nanotubes are lightweight in nature and are used to produce lightweight and biodegradable nanocomposite foams. 160 The structural parameters of carbon nanotubes define whether they will be semiconducting or metallic in nature. This property of carbon nanotubes is considered to be effective for their use as a central element in the design of electronic devices such as rectifying diodes, 161 single-electron transistors, 162 and field-effect transistors. 163 The chemical stability, nano-size, high electrical conductivity, and amazing structural perfection of carbon nanotubes make them suitable for electron field emitter applications. 164 The unique set of mechanical and electrochemical properties make CNTs a valuable smart candidate for use in lithium-ion batteries. 165 CNTs have the full potential to be used as a binderless free-standing electrode for active lithium-ion storage. CNT-based anodes can have reversible lithium-ion capacities exceeding 1000 mA h g −1 , and this is a substantial improvement compared with conventional graphite anodes. In short, the following factors play a role in controlling and optimizing the performances of CNT-based composites: 166 (i) the volume fraction of carbon nanotubes; (ii) the CNT orientation; (iii) the CNT matrix adhesion; (iv) the CNT aspect ratio; and (iv) the composite homogeneity.
For some applications, a proper stable aqueous dispersion of CNTs at a high concentration is pivotal to allow the system to perform its function efficiently and effectively. 167 One of the major issues associated with carbon nanotubes is their poor dispersion in aqueous media due to their hydrophobic nature. Clusters of CNTs are formed due to van der Waals attraction, π–π stacking, and hydrophobicity. The CNT clusters, due to their strong interactions, hinder solubility or dispersion in water or even organic-solvent-based systems. 168 This challenging dispersion associated with CNTs has limited their use for promising applications, such as in biomedical devices, drug delivery, cell biology, and drug delivery. 167 Carbon nanotube applications and inherent characteristics can be further tuned via suitable functionalization. The functionalization of carbon nanotubes helps scientists to manipulate the properties of carbon nanotubes and, without functionalization, some properties are not attainable. 169 The functionalization of nanotubes can be divided into two main categories: covalent functionalization and non-covalent functionalization.
The heating of CNTs under strongly acidic and oxidative conditions results in the formation of oxygen-containing functionalities. These functional groups, such as carboxylic acid, react further with other functional groups, such as amines or alcohols, to produce amide or ester linkages on the carbon nanotubes. 172 One of the main issues preventing the utilization of CNTs for biomedical applications is their toxicity. The cytotoxicity of pristine carbon nanotubes can be reduced via introducing carbonyl, –COOH, and –OH functional groups. Apart from functionalization through oxidized CNTs, the direct functionalization of CNTs is also possible. However, direct functionalization requires more reactive species to directly react with the CNTs, such as free radicals. Addition reactions to CNTs can cause a transformation from sp 2 hybridization to sp 3 hybridization at the point of addition. At the point where functionalization has taken place, the local bond geometry is changed from trigonal planar to tetrahedral geometry. Some addition reactions to the sidewalls of CNTs are shown in Fig. 16 . 155
It is important to discuss how the covalent functionalization of carbon nanotubes comes at the price of the degradation of the carbon sp 2 network. This substantially affects the electronic, thermal, and optoelectronic properties of the carbon nanotubes. 169 Efforts are being made to introduce a new method of covalent functionalization that can keep the π network of CNTs intact. Antonio Setaro et al. introduced a new [2+1] cycloaddition reaction for the non-destructive, covalent, gram-scale functionalization of single-walled carbon nanotubes. The reaction rebuilds the extended π-network, and the carbon nanotubes retained their outstanding quantum optoelectronic properties ( Fig. 17 ). 173
Polymers are frequently combined with CNTs to enhance their dispersion capabilities. Polymers interact with CNTs through CH–π and π–π interactions. 174 Hexanes and cycloalkanes are poor CNT solvents but the good solubility or dispersion of CNTs in these solvents is required for surface coating applications. Poly(dimethylsiloxane) (PDMS) macromer-grafted polymers have been prepared using PDMS macromers and pyrene-containing monomers that strongly adsorb on CNTs, thus improved the solubility of CNTs in chloroform and hexane. 176 The use of head–tail surfactants is another attractive way to achieve a fine dispersion of CNTs in an aqueous medium. In head–tail surfactants, the tail is hydrophobic and interacts with the CNT sidewalls, and the hydrophilic head groups interact with the aqueous environment to provide a fine dispersion. 177
For electrical applications, non-covalently functionalized CNTs are more preferred because the electrical properties of the CNTs are not compromised. CNTs have been non-covalently functionalized with a variety of biomolecules for the fabrication of electrochemical biosensors. 175 Non-covalently functionalized SWCNTs are used for energy applications. Single-walled carbon nanotubes (SWCNTs) have been non-covalently functionalized with 3d transition metal( II ) phthalocyanines, lowering the potential of the oxygen evolution reaction by approximately 120 mV compared with unmodified SWCNTs. 178 The toxicity of pristine CNTs toward living organisms can be lessened via using surfactant-functionalized CNTs. 170 However, in some cases, during polymer non-covalent functionalization, the polymer may wrap CNT bundles and make it difficult to separate the CNTs from each other. Polymers can develop into insulating wrapping that affects the CNT conductivity.
In the literature, several graphene-related materials have been reported, such as graphene oxide and reduced graphene oxide. 187 Among graphenoids, graphene oxide is a more reported and explored graphene-related material as a precursor for chemically modified graphene. The synthetic route to graphene oxide is straightforward, and it is synthesized from inexpensive graphite powder that is readily available. 188 Graphene oxide has many oxygen-containing functional groups, such as epoxy, hydroxyl, carboxyl, and carbonyl groups. The basal plane of graphene oxide is generally decorated with epoxide and hydroxyl groups, whereas the edges presumably contain carboxyl- and carbonyl-based functional groups. 189 The presence of active functional groups in graphene oxide allows its further functionalization with different polymers, small organic compounds, or other nanomaterials to realize several applications. 190
Graphene oxide, due to its oxygen functionality, is insulating in nature and displays poor electrochemical performance. The presence of oxygen functionalities in graphene oxide breaks the conjugated structure and localizes the π-electron network, resulting in poor carrier mobility and carrier concentration. 196 Its electrochemical performance is improved substantially after removing the oxygen-containing functional groups. 197 These functional groups can be removed or reduced via thermal, electrochemical, and chemical means. The product obtained after removing or reducing oxygen moieties is called reduced graphene oxide. The properties of reduced graphene oxide depend upon the effective removal of oxygen moieties from graphene oxide. The process used to remove oxygen-containing functionalities from graphene oxide will determine the extent to which reduced the properties of graphene oxide resemble pristine graphene. 198
Reduced graphene oxide is extensively used to improve the performances of various electrochemical devices. 199 It is essential to mention that even after reducing graphene oxide, some residual sp 3 carbon bonded to oxygen still exists, which somehow disturbs the movement of charge through the delocalized electronic cloud of the sp 2 carbon network. 200 Apart from this, the electrochemical activity of reduced graphene oxide is substantially high enough to manufacture electrochemical devices with improved performances. Recently, the demand for super-performance electrochemical devices has increased to overcome modern challenges relating to electronics and energy-storage devices. 201 Graphene-based materials are considered to be excellent electrode materials, and they can be proved to be revolutionary for use in energy-storage devices such as supercapacitors (SC) and batteries. Graphene-based electrodes improve the performances of existing batteries (lithium-ion batteries) and they are considered useful for developing next-generation batteries such as sodium-ion batteries, lithium–O 2 batteries, and lithium–sulfur batteries ( Fig. 18 ). Being flat in nature, each carbon atom of graphene is available, and ions can easily access the surface due to low diffusion resistance, which provides high electrochemical activity. 202
Graphene and its derivatives are extensively used for the development of electrochemical sensors. 203 The surfaces of bare electrodes are usually not able to sense analytes at trace levels and they cannot differentiate between analytes that have close electrooxidation properties due to their poor surface kinetics. The addition of graphene layers to the surfaces of electrodes can substantially improve the electrocatalytic activity and surface sensitivity towards analytes. 204 Graphene has definite advantages over other materials that are used as electrode materials for sensor applications. Graphene has a substantially high surface-to-volume ratio and atomic thickness, making it extremely sensitive to any changes in its local environment. This is an essential factor in developing advanced sensing tools, as all the carbon atoms are available to interact with target species.
Consequently, graphene exhibits higher sensitivity than its counterparts such as CNTs and silicon nanowires. 205 Graphene has two main advantages over CNTs for the development of electrochemical sensors. First, graphene is mostly produced from graphite, which is a cost-effective route, and second, graphene does not contain metallic impurities like CNTs can. Graphene offers many other advantages when developing sensors and biosensors, such as biocompatibility and π–π stacking interactions with biomolecules. 206 Graphene-based materials are ideal for the construction of nanostructured sensors and biosensors.
The mechanical properties of graphene are used to fabricate highly desired stretchable and flexible sensors. 207 Graphene can be utilized to develop transparent electrodes with excellent optical transmittance. It displays good piezoresistive sensitivity. Researchers are making efforts to replace conventional brittle indium tin oxide (ITO) electrodes with flexible graphene electrodes in optoelectronic devices such as liquid-crystal displays and organic light-emitting diodes. 208 For human–machine interfaces, transparent and flexible tactile sensors with high sensitivity have become essential. Graphene film (GF) and PET have been applied to develop transparent tactile sensors that exhibit outstanding cycling stability, fast response times, and excellent sensitivity ( Fig. 19 ). 209 Similarly, graphene is applied for the fabrication of pressure sensors. 210 Overall, graphene is an excellent material for developing transparent and flexible devices. 211,212
The use of graphene-based materials is an effective way to deal with a broad spectrum of pollutants. 213 There are many ways to deal with environmental pollution; among these, adsorption is an effective and cost-effective method. 214,215 Graphene-based adsorbents are found to be useful in the removal of organic, 216 inorganic, and gaseous contaminants. Graphene-based materials have some obvious advantages over CNT-based adsorbents. For example, graphene sheets offer two basal planes for contaminant adsorption, enhancing their effectiveness as an adsorbent. 192 GO contains several oxygen functional groups that impart hydrophilic features. Due to appropriate hydrophilicity, GO-based adsorbents can efficiently operate in water to remove contaminants. Moreover, graphene-oxide-based materials can be functionalized further through reactive moieties with various organic molecules to enhance their adsorption capacities. 217
In short, extensive research must continue in order to develop graphene-based materials with high performance and bring them to the market. Massive focus on graphene research is also justified due to the extraordinary features described in extensive theoretical and experimental research works.
Nanodiamonds possess a core–shell-like structure and display rich surface chemistry, and numerous functional groups are present on their surface. Several functional groups, such as amide, aldehyde, ketone, carboxylic acid, alkene, hydroperoxide, nitroso, carbonate ester, and alcohol groups, are present on nanodiamond surfaces, assisting in their further functionalization for desired applications ( Fig. 20 ). 226
Furthermore, nanodiamond surfaces can be homogenized with a single type of functional group according to the application requirements. 227 The use of nanodiamond particles as a reinforcing material in polymer composites has attracted great attention for improving the performance of polymer composite materials. The superior mechanical properties and rich surface chemistry of nanodiamonds have made them a superior material for tuning and reinforcing polymer composites. Nanodiamonds might operate via changing the interphase properties and forming a robust covalent interface with the matrix. 228 Nanodiamond (ND)-reinforced polymer composites have shown superior thermal stabilities, mechanical properties, and thermal conductivities. Nanodiamonds have shown great potential for energy storage applications. 229 Nanodiamonds and their composites are also used in sensor fabrication, environmental remediation, and wastewater treatment. 230,231 Their stable fluorescence and long fluorescence lifetimes have made nanodiamonds useful for imaging and cancer treatment. For biomedical applications, the rational engineering of nanodiamond particle surfaces has played a crucial role in the carrying of bioactive substances, target ligands, and nucleic acids, resisting their aggregation. 232,233 Nanodiamonds have a great future in nanotechnology due to their amazing surface chemistry and unique characteristics.
Carbon quantum dots can be synthesized through several chemical routes. 241–245 Some methodologies for synthesizing carbon dots are described in Fig. 21 . 246–248 Carbon itself is a black material and displays low solubility in water. In contrast, carbon quantum dots are attractive due to their excellent solubility in water. They contain a plethora of oxygen-containing functional groups on their surface, such as carboxylic acids. These functional moieties allow for further functionalization with biological, inorganic, polymeric, and organic species.
Carbon quantum dots are also called carbon nano-lights due to their strong luminescence. 248 In particular, carbon quantum dots offer enhanced chemiluminescence, 249,250 fluorescent emission, 251 two-photon luminescence under near-infrared pulsed-laser excitation, 252 and tunable excitation-dependent fluorescence. 253 The luminescence characteristics of carbon quantum dots have been used to develop highly sensitive and selective sensors. In most cases, a simple principle is involved in sensing with luminescent carbon quantum dots: their photoluminescence intensity changes upon the addition of an analyte. 254 Based on this principle, several efficient sensors have been developed using carbon quantum dots. 255–257 They can be used as sensitive and selective tools for sensing explosives such as TNT. Recognition molecules on the surfaces of carbon quantum dots can help to sense targeted analytes. Amino-group-functionalized carbon quantum dot fluorescence is quenched in the presence of TNT through a photo-induced electron-transfer effect between TNT and primary amino groups. This quenching phenomenon can help to sense the target analyte ( Fig. 22 ). 258 Chiral carbon quantum dots (cCQDs) can exhibit an enantioselective response. The PL responses of cCQDs were evaluated toward 17 amino acids and it was found that the PL intensity of the cCQDs was only substantially enhanced in the presence of L -Lys ( Fig. 22 ). 254
Carbon quantum dots have received significant interest in the fields of biological imaging and nanomedicine ( Fig. 23 ). 239 Direct images of RNA and DNA are essential for understanding cell anatomy. Due to the limitations of current imaging probes, tracking the dynamics of these biological macromolecules is not an easy job. Recently, membrane-penetrating carbon quantum dots have been developed for the imaging of nucleic acids in live organisms. 259 It is important to note that most of the carbon quantum dots utilized to attain cell imaging under UV excitation emit blue radiation. Some biological tissue also emits blue light, specifically that involving carbohydrates, and this interferes with cell imaging carried out with blue-emitting CQDs. This seriously hinders their potential in the field of biomedical imaging. Due to this reason, researchers are focusing on tuning CQDs in a way that their emission peak is red-shifted to avoid interference. 260 Carbon quantum dots with yellow and green fluorescence have been reported for bioimaging purposes. 261,262 The suitable doping of carbon quantum dots can red-shift the emission to enhance the bioimaging effectiveness. 263 Doped carbon quantum dots are capable of biological imaging and display advanced capabilities for scavenging reactive oxygen species. 264
Carbon quantum dots demonstrate photo-induced electron transfer properties 265 that make them valuable for photocatalytic, light-energy conversion, and other related applications. 266 Carbon quantum dots enhance the activities of other photocatalysts to which they are attached. Carbon quantum dots, along with photocatalysts, provide better charge separation and suppress the regeneration of photogenerated electron–hole pairs. Moreover, the proper implantation of carbon quantum dots into photocatalysts can broaden the photo-absorption region. Implanted carbon quantum dots form micro-regional heterostructures that facilitate photo-electron transport. 267 The implantation of carbon quantum dots into g-C 3 N 4 can substantially enhance charge transfer and separation efficiencies, prevent photoexcited carrier recombination, narrow the bandgap, and red shift the absorption edge. 268 The intrinsic catalytic activity of polymeric carbon nitride is improved as a result of the nano-frame heterojunctions formed with the help of CQDs. 269
Carbon quantum dots offer many advantages over conventional semiconductor-based QDs and, thus, they have attracted considerable researcher attention. 244 Due to their remarkable features, they have shown importance in recent years in the fields of light-emitting diodes, nanomedicine, solar cells, sensors, catalysis, and bioimaging. 236
The production of carbon nanohorns has some obvious advantages over carbon nanotubes, such as the ability for toxic-metal-catalyst-free synthesis and large-scale production at room temperature. Carbon nanotube synthesis involves metal particles, and harsh conditions, such as the use of strong acids, are required to remove metallic catalysts. This process introduces many defects into CNT structures and may cause a loss of carbon material. 270 Carbon nanohorns possess a wide diameter compared to CNTs. CNHs possess good absorption capabilities and their interiors are also available after partial oxidation, which provides direct access to their internal parts. Heat treatment under acidic or oxidative conditions facilitates the facile introduction of holes into carbon nanohorns. Holes in graphene sheets of single-walled carbon nanohorns can be produced with O 2 gas at high temperatures. A large quantity of material can be stored inside CNH tubes. 274 The surface area of CNHs is substantially enhanced upon opening the horns to make their interiors accessible. 275 Carbon nanohorns have great potential for energy storage, 275 electrochemiluminescence, 276 adsorption, 277 catalyst support, 278 electrochemical sensing, 279 and drug delivery system 273 uses. CNHs as catalyst supports can provide a homogeneous dispersion of Pt nanoparticles ( Fig. 25 ). The current density of Pt supported on single-walled carbon nanohorns is double compared to a fuel cell made from Pt supported on carbon black. 280 Thus, carbon nanohorns provide a better uniform dispersion that facilitates a high surface area and better catalyst performance.
5.2. Nanoporous materials
In nanoporous materials, the size distributions, volumes, and shapes of the pores directly affect the performances of porous materials for particular applications. It has become a hot area of research to develop materials with precisely controlled pores and arrangements. Recent research has focused more on the precise control of the shapes, sizes, and volumes of pores to produce nanoporous materials with high performance. Several state-of-the-art reviews are present in the literature that focus explicitly on the synthesis, properties, advances, and applications of nanoporous materials. 85,287–289 Based on the materials used, nanoporous materials can be divided into three main groups: inorganic nanoporous materials; carbonaceous nanoporous materials; and organic polymeric nanoporous materials.
Inorganic nanoporous materials include porous silicas, clays, porous metal oxides, and zeolites. The generation of pores in the material can introduce striking features into the material that are absent in non-porous materials. Nanoporous materials offer rich surface compositions with versatile characteristics. Nanoporous materials exhibit high surface-to-volume ratios. Their outstanding features and nanoporous framework structures have made these materials valuable in the fields of environmental remediation, adsorption, catalysis, sensing, energy conversion, purification, and medicine. 284,290
Porous silica is a crucial member of the inorganic nanoporous family. Over the decades, it has generated significant research interest for use in fuel cells, chemical engineering, ceramics, and biomedicine. It is essential to note that specific morphologies and pore size diameters are required for each application, and these can be achieved via tuning during the synthesis process. Nanoporous silica offers two functional surfaces: one is the cylindrical pore surfaces, and the second is the exterior surfaces of the nanoporous silica particles. The surfaces of nanoporous silica can be easily functionalized for the desired applications. The nanoporous silica surface is heavily covered with many silanol groups that act as reactive sites for functionalization ( Fig. 26 ). 291,292 For biomedical applications, mesoporous silica has emerged as a new generation of inorganic platform materials compared to other integrated nanostructured materials. Several factors make it a unique material for biomedical applications: 293,294 (a) its ordered porous structure; (b) its tunable particle size; (c) its large pore volume and surface area; (d) its biocompatibility; (e) its biodegradation, biodistribution, and excretion properties; and (f) its two functional surfaces. For instance, ordered MCM-48 nanoporous silica was used for the delivery of the poorly soluble drug indomethacin. It has been found that surface modification can control drug release. 295 Mesoporous silica-based materials have emerged as excellent materials for use in sustained drug delivery systems (SDDSs), immediate drug delivery systems (IDDSs), targeted drug delivery systems (TDDSs), and stimuli-responsive controlled drug delivery systems (CDDSs). The drug release rate from mesoporous silica can also be controlled via introducing appropriate polymers or functional groups, such as CN, SH, NH 2 , and Cl. Researchers are currently focusing on developing MSN-based (MSN = mesoporous silica nanoparticle) multifunctional drug delivery systems that can release antitumor drugs on demand in a targeted fashion via minimizing the premature release of the drug ( Fig. 27 ). 296
Hierarchically nanoporous zeolites are a vital member of the nanoporous material family. They are crystalline aluminosilicate minerals whose structures comprise uniform, regular arrays of nanopores with molecular dimensions. The microporous structures of zeolites contain pores that are usually below 1 nm in diameter. In zeolites, the micropores are uniform in shape and size, and these pores can effectively discriminate between molecules based on shape and size. 297 Currently, based on crystallography, more than 200 zeolites have been classified. 298 Zeolites have been proved to be useful materials in the field of host–guest chemistry. In solid catalysis, about 40% of the entire solid catalyst field is taken up by zeolites in chemical industry. The excellent catalysis success of zeolites is based on their framework stability, shape-selective porosity, solid acidity, and ion-exchange capacity. Oxygen tetrahedrally coordinates with the Al atoms in most zeolite crystalline silicate frameworks, resulting in charge mismatch between the oxide framework and Al. Extra-framework Na + ions compensate for this charge mismatch. The Na + ions are exchangeable for other cations such as H + and K + . 298 The zeolite crystalline networks are remarkable in that they provide high mechanical and hydrothermal stabilities. The most crucial task facing the zeolite community is to find new structures with desired functions and apply them more effectively for different applications.
Apart from these inorganic porous materials, several other metal- and metal-oxide-based nanoporous materials have been introduced that are more prominent for use in electrode material, catalyst, photodegradation, energy storage, and energy conversion applications. 299–302 Nanoporous metal-based materials are famous due to the nanosized crystalline walls, interconnected porous networks, and numerous surface metal sites that provide them with unique physical/chemical properties compared with their bulk counterparts and other nanostructured materials. 303 For example, nanoporous WO 3 films were developed via tuning the anodization conditions for photoelectrochemical water oxidation. It has been observed that the morphology of the film strongly affected the photoelectrochemical performance. 304 Nanoporous alumina is also a unique material in the inorganic nanoporous family due to several aspects. Nanoporous alumina can be prepared in a controlled fashion with any size and shape in polyprotic aqueous media via the anodic oxidation of the aluminum surface. The parallel arrangement of pores on alumina can easily be controlled from 5 nm to 300 nm, and alumina is stable in the range of 1000 °C. The anodizing time plays a significant role in controlling the pore length. Nanoporous alumina membranes offer various unique properties, such as pores of variable widths/lengths, temperature stability, and optical transparency. Nanoporous alumina pores can be filled with magnetically and optically active elements to produce the desired applications at the nanoscale level. Photoluminescent alumina membranes can be produced via introducing cadmium sulfide, gallium nitride, and siloxenes inside nanoporous alumina using appropriate precursors. 305 Porous alumina also acts as an efficient support and template for the designing of other nanomaterials. Palladium nanowires, 306 high aspect ratio cobalt nanowires, 307 and highly aligned Cu nanowires 308 were developed using porous alumina as a template. Ni–Pd as a catalyst was supported on porous alumina for hydrogenation and oxidation reactions. 309 Nanoporous anodic alumina is also considered to be an efficient material for the development of biosensors due to the ease of fabrication, tunable properties, optical/electrochemical properties, and excellent stability in aqueous environments. 310
Nanoporous carbon-based materials are a hot topic in the field of materials chemistry. Nanoporous carbon materials have become ubiquitous choices in the environmental, energy, catalysis, and sensing fields due to their unique morphologies, large pore volumes, controlled porous structures, mechanical, thermal, and chemical stabilities, and high specific surface areas ( Fig. 28A ). 311 Nanoporous materials are found to be useful in the treatment of water. The separation of spilled oil and organic pollutants from water has emerged as a significant challenge. 312–314 The design of materials that can allow the efficient separation of organic, dye, and metal contaminants from water has become a leading environmental research area. 315,316 Nanoporous carbon can be derived from different natural and synthetic sources. 317–319 Nanoporous carbon foam can be derived from natural sources, such as flour, pectin, and agar, via table-salt-assisted pyrolysis. The agar-derived nanoporous carbon foam showed high absorption capacities, a maximum of 202 times its own weight, for oil and organic solvents. Air filtration paper developed from carbon nanoporous materials and non-woven fabrics has shown a filtration efficiency of greater than 99% ( Fig. 28B ). 320 Nanoporous carbon can also be produced from other porous frameworks, such as metal–organic frameworks. MOF- and COF-based materials are promising precursors for nanoporous carbon-based materials. The direct carbonization of amino-functionalized aluminum terephthalate metal–organic frameworks has produced nitrogen-doped nanoporous carbon that shows an adequate removal capacity of 98.5% for methyl orange under the optimum conditions. 321 Fe 3 O 4 /nanoporous carbon was also produced with Fe salts as a magnetic precursor and MOF-5 as a carbon precursor for removing the organic dye methylene blue (MB) from aqueous solutions. 322 The mesoporous carbon removal efficiency could be further enhanced via modifying or functionalizing the surface with various materials. Unmodified mesoporous carbon has shown a mercury removal efficiency of 54.5%. This efficiency can be substantially improved to 81.6% and 94% upon modification with the anionic surfactant sodium dodecyl sulfate and cationic surfactant cetyltrimethyl ammonium bromide (CTAB), respectively. 323
Ordered nanoporous carbon, CNTs, and fullerenes are extensively applied for energy and environmental applications. The complicated synthesis routes required for fullerenes and CNTs have slowed down the full exploitation of their potential for highly demanding applications. In comparison, the synthesis of highly ordered nanoporous carbon is facile, and the properties of ordered nanoporous carbon are also appealing for energy and environmental applications. 311 CO 2 is a greenhouse gas, and its sustainable conversion into value-added products has become the subject of extensive research. A nitrogen-doped nanoporous-carbon/carbon-nanotube composite membrane is a high-performance gas-diffusion electrode applied for the electrocatalytic conversion of CO 2 into formate. A faradaic efficiency of 81% was found for the production of formate. 324 Nanoporous carbon materials modified with the non-precious elements P, S, N, and B have emerged as efficient electrode materials for use in the oxygen evolution reaction (OER), hydrogen evolution reaction (HER), oxygen reduction reaction (ORR), batteries, and fuel cells. 311,325–327
Nanoporous polymers, including nanoporous coordination polymers and crystalline nanoporous polymers, have emerged as impressive nanoporous materials. 328 Nanoporous polymers have many applications, and these materials are extensively being evaluated for gas separation and gas storage. The great interest in these applications arises from the presence of pores providing an exceptionally high Brunauer–Emmett–Teller (BET) surface area. Recently, new classes of metal organic framework and covalent organic framework porous materials have been reported that have shown exceptionally high and unprecedented surface areas. For instance, in 2010, a MOF was reported with a surface area of 6143 m 2 g −1 ; 329 in 2012, a MOF was reported with a surface area greater than 7000 m 2 g −1 ; 330 and in 2018, a MOF (DUT-60) was reported with a record surface area of 7836 m 2 g −1 . 331 Mesoporous DUT-60 has also shown a high free volume of 90.3% with a density of 0.187 g cm −3 . 331
Due to their exceptionally high surface areas and porous networks, these MOFs and COFs are ideal for gas storage. Air separation and post-combustion CO 2 capture have become integral parts of mainstream industries related to the energy sector in order to avoid substantial economic penalties. Due to the inefficiencies of available technology and the critical importance of this area, earnest efforts are being made to design gas-selective porous materials for the selective adsorption of desired gases. Nanoporous MOF- and COF-based materials can significantly capture CO 2 and help reach zero or minimum CO 2 emission levels. For instance, nanoporous fluorinated metal–organic frameworks have shown the selective adsorption of CO 2 over H 2 and CH 4 . 332 Hasmukh A. Patel et al. developed N 2 -phobic nanoporous covalent organic polymers for the selective adsorption of CO 2 over N 2 . The azo groups in the framework rejected N 2 , leading to CO 2 selectivity. 333 Nanoporous polymers that are superhydrophobic in nature can also be used for volatile organic compounds and organic contaminants. 334 Nanoporous polymers, due to the presence of a porous network, have been considered as highly suitable materials for catalyst supports. Furthermore, organocatalytic functional groups can be introduced pre-synthetically and post-synthetically into solid catalysts. 335
Nanoporous polymeric materials are amazingly heading towards being extremely lightweight with exceptionally high surface areas. These high surface areas and the fine-tuning of the nanopores has made these nanoporous materials, specifically MOFs and zeolites, ideal support materials for encapsulating ultrasmall metal nanoparticles inside void spaces to produce nanocatalysts with exceptionally high efficiencies. 336 In the coming years, more exponential growth of nanoporous materials is expected in the energy, targeted drug delivery, catalysis, and water treatment fields.
5.3. Ultrathin two-dimensional nanomaterials beyond graphene
However, from a material synthesis standpoint, a graphite-like layered form of Si does not exist in nature and there is no conventional exfoliation process that can generate 2D silicene, although single-walled 351 and multi-walled 352 silicon nanotubes and even monolayers of silicon have been synthesized via exfoliation methods. 353 Forming honeycomb Si nanostructures on substrates like Ag(001) and Ag(110) via molecular beam deposition, so-called “epitaxial growth”, was then proposed as a method for the architectural design of silicene sheets. 354–356 The successful synthesis of a silicene monolayer was first achieved on Ag(111) and ZrB 2 (0001) substrates in 2012; 357,358 later, various demonstrations were made using Ir(111), ZrB 2 (001), ZrC(111), and MoS 2 surfaces as the silicene growth substrates. 359–361 Despite various extensive studies to date involving the “epitaxial growth” of silicene on different substrates and investigations of the electronic properties, 357,362–364 the limited nanometer size, difficulties relating to substrate removal, and air stability issues have substantially impeded the practical applications of silicene. Bearing in mind all these known difficulties, Akinwande and co-workers recently reported a growth–transfer–fabrication process for novel silicene-based field-effect transistor development that involved silicene-encapsulated delamination with native electrodes. 365 An etch-back approach was used to define source/drain contacts in Ag film. Without causing any damage to the silicene, a novel potassium-iodide-based iodine-containing solution was used to etch Ag, avoiding rapid oxidation, unlike other commonly used Ag etchants. The results demonstrated that this was the first proof-of-concept study confirming the Dirac-like ambipolar charge transport predictions made about silicene devices. Comparative studies with a graphene system, the low residual carrier density, and the high gate modulation suggested the opening of a small bandgap in the experimental devices, proving that silicene can be considered a viable 2D nanomaterial beyond graphene.
Nonetheless, the synthesis of silicene on a large-scale is greatly limited, as “epitaxial growth” is the only promising method for obtaining high-quality silicene, and this presents an enduring challenge in relation to silicene research and development. Xu and co-workers recently introduced liquid oxidation and the exfoliation of CaSi 2 as a means for the first scalable preparation of high-quality silicene nanosheets. 366 This new synthetic strategy successfully induced the exfoliation of stacked silicene layers via the mild oxidation of the (Si 2 n ) 2 n layers in CaSi 2 into neutral Si 2 n layers without damage to the pristine silicene structure ( Fig. 29 ). The selective oxidation of pristine CaSi 2 into free-standing silicene sheets without any damage to the original Si framework was carried out via exfoliation in the presence of I 2 in acetonitrile solvent. Furthermore, the obtained silicene sheets yielded ultrathin monolayers or layers with few-layer thickness and exhibited excellent crystallinity. This 2D silicene nanosheet material was extensively explored as a novel anode, which was unlike previously developed silicon-based anodes for lithium-ion batteries. It displayed a theoretical capacity of 721 mA h g −1 at 0.1 A g −1 and superior cycling stability of 1800 cycles. Overall, during the last decade, silicene has been widely accepted as an ideal 2D material with many fascinating properties, suggesting great promise for a future beyond graphene.
Like other 2D materials, MXenes exhibit crystal geometry with a hexagonal close-packed structure based on the equivalent MAX-phase precursor, and the close-packed structure is formed from M atoms with X atoms occupying octahedral sites. 371 According to the formula, there are three representative structures of MXenes: M 2 XT x , M 3 X2T x , and M 4 X3T x . In these combinations, X atoms are formed with n layers, whereas M atoms have n + 1 layers ( Fig. 30 ). 372 Apart from graphene, MXenes are considered the most dynamic developing material, and they have incredible innovation potential amongst typical 2D nanomaterials because of their remarkable properties, such as hydrophilicity, conductivity, considerable adsorption abilities, and catalytic activity. These vital properties of MXenes suggest their use for various potential applications, including in the photocatalysis, electrocatalysis, 373,374 energy, 375 membrane-based separation, 376,377 and biological therapy 378 fields. In this section, we focus on describing new developments relating to MXenes that are utilized for electrocatalytic and energy storage applications, competing as alternatives to graphene materials.
Interestingly, due to the presence of abundant terminal groups, mainly –O, –OH, and –F, and their modifying nature, MXenes can exhibit outstanding hydrophilic properties and high conductivity and charge carrier mobility, making them a very attractive material for various electrocatalytic applications, such as the hydrogen evolution reaction, oxygen evolution reaction, oxygen reduction reaction, nitrogen reduction reaction, and CO 2 reduction reaction. To further increase their electrocatalytic activities, recent works involving MXenes have included incorporation with CNTs, 379 g-C 3 N 4 , 380 FeNi-LDH, 381 NiFeCo-LDH, 382 and metal–organic frameworks. 383
Cho and co-workers designed and developed MXene–TiO 2 2D nanosheets via the surface oxidation of MXene with defect-free control. These MXene–TiO 2 2D nanosheets were successfully implemented in nano-floating-gate transistor memory (NFGTM) providing a floating gate ( i.e. , multilayer MXene) and tunneling dielectric ( i.e. , the TiO 2 layer). A process of oxidation in water further represented a cost-effective and environmentally benign method, as depicted in Fig. 31 . The MXene NFGTM with an optimal oxidation process displayed exceptional nonvolatile memory features, having a great memory window, high programming/erasing current ratio, long term retention, and high durability. 384
There have been some exciting reports on 2D materials from the pnictogen family, particularly phosphorene. Recently, more attention has also been given to the remaining group 15 elements, 390 with the novel 2D materials arsenene, antimonene, and bismuthene being obtained from the key elements arsenic, antimony, and bismuth, respectively. It is reported that 2D monolayers of group 15 elements, including phosphorene allotropes, have five distinct honeycomb (α, β, γ, δ, and ε) and four distinct non-honeycomb (ζ, η, θ, and ι) structures, as depicted in Fig. 32 . Dissimilar crystal orientations were found for single-layered As, Sb, and Bi. Zeng and co-workers also reported comprehensive density functional theory (DFT) computations that proved the energetic stability and broad-range application of these materials in 2D semiconductors. 391 Previously, following theoretical predictions, Wu and co-workers successfully demonstrated that α-phosphorene showed lowest energy configurations in both honeycomb and non-honeycomb nanosheets. 392 In contrast, Zeng and co-workers proved that the buckled forms of 2D sheets of As, Sb, and Bi allotropes are the most stable structures, particularly their β phases. 391
Among monolayer group 15 family materials, 2D sheets of arsenic (As) and antimony (Sb) have gained considerable attention from researchers. 393,394 Studies have shown that As and Sb exhibit better stability than black phosphorus; they are highly stable at room temperature and less reactive to air, likely inhibiting the oxidization process. 395–398 Nevertheless, it has been demonstrated that the oxidation process is perhaps favorable for fine-tuning the electronic properties; increases in the indirect band gaps ranging from 0 to a maximum of 2.49 eV are found in free-standing arsenene and antimonene semiconductors. 399–403 Simultaneously, arsenene and antimonene can also be transformed into semiconductors with direct band gaps. These two 2D nanosheets can be used to design mechanical sensors, moving beyond common electronic and optoelectronic applications. These two extraordinary 2D nanosheets have been studied for their structural–property relationships via first-principles methods. 403–405
Continuing the characterization and structural property studies of arsenene carried out by Kamal 404 et al. and Zhang 403 et al. , Anurag Srivastava and co-workers analyzed applications of arsenene to explore the possibility of improving sensor devices that can be utilized to detect ammonia (NH 3 ) and nitrogen dioxide (NO 2 ) molecules. 406,407 They investigated the affinities of NH 3 and NO 2 molecules for pristine arsenene sheets, examining the binding energies, bonding distances, density distributions, and current–voltage features. The results showed that arsenene 2D sheets are highly durable, with significant electronic charge transfer. They also considered germanium-doped arsenene and characterized the 2D lattice based on molecular affinity relationships with respect to the dopant.
However, the incorporation of any dopants into 2D nanomaterials not only results in experimental difficulty but it also lowers the stability of 2D materials. 408 Recently, Dameng Liu and co-workers reported the electronic structures, focusing on band structures, band offsets, and intrinsic defect properties, of few-layer arsenic and antimony. 409 The spontaneous oxide passivation layer that is formed naturally on pristine antimonene provides excellent stability. 410 Very recently, Stefan Wolff and co-workers conducted DFT calculations on various single or few-layer antimony oxide structures to describe the stoichiometry and bonding type. Interestingly, the samples exhibited various structural stabilities and electronic properties with a wide range of direct and indirect band gaps. Showing band gaps between 2.0 and 4.9 eV, these 2D layers of antimonene exhibited the potential to be used as insulators or semiconductors. 411 The same group also analyzed Raman spectra and discussed identifying the predicted antimonene oxide structures experimentally. The enduring task of exploring the utility of antimonene has boosted recent research interest in 2D nanomaterials due to the broad range of potential applications, such as their use in electrochemical sensors, 412,413 stable organic solar cells, 414 and supercapacitors 415 to name a few.
The 2D MOF nanosheets are also evaluated for the development of high-performance power-storage devices. For example, Li et al. 427 recently reported two novel Mn-2D MOFs and Ni-2D MOFs as anode materials for rechargeable lithium batteries. The Mn-based ultrathin metal–organic-framework nanosheets, due to thinner nanosheets, a higher specific surface area, and smaller metal ion radius, had structural advantages over Ni-based ultrathin metal–organic-framework nanosheets. Due to these features, the Mn-based ultrathin metal–organic-framework nanosheets displayed a high reversible capacity of 1187 mA h g −1 at 100 mA g −1 for 100 cycles and a rate capability of 701 mA h g −1 even at 2 A g −1 .
The expensive metal oxides utilized in the catalytic process can be replaced in due course by 2D-MOF-based nanosheets with exposed metal sites that impart an adjustable pore structure, ultrathin thickness, a high surface-to-volume atom ratio, and high design flexibility. As a result, 2D-MOFs have extensively been explored for various electrocatalytic applications, including the hydrogen evolution reaction (HER), oxygen evolution reaction (OER), oxygen reduction reaction (ORR), and carbon dioxide reduction reaction (CO 2 RR). For example, Marinescu et al. 428 combined cobalt dithiolene species with benzenehexathiol (BHT) and yielded 2D-MOFs capable of acting as electrocatalysts for the HER in water ( Fig. 34 ). In the presence of 2D-MOF sheets, a high current density of 41 mA cm −2 , at −0.8 V vs. SHE and a pH value of 1.3, is observed. Similarly, Feng et al. 429 also developed single-layer Ni-based 2D-MOF sheets that are highly effective for electrocatalytic hydrogen evolution. Later, Patra et al. 430 reported similar 2D sheets from covalent organic frameworks (2D-COFs) as metal-free catalysts for HER applications. 2D-MOFs are also being explored as active catalysts for the OER process. For example, Xu et al. 431 reported the preparation of 2D Co-MOF sheets using polyvinylpyrrolidone as a surfactant under mild solvothermal conditions. These novel 2D Co-MOFs displayed ultrathin nanosheets with many surface-based metal active sites, improving the overall OER performance.
Interestingly, experimental electrochemical measurement data showed that Co-MOF sheets offer a low overpotential ( i.e. , 263 mV at 10 mA cm −2 ). Similarly, Wang et al. 432 also reported that double-metal 2D-sheets (2D NiFe MOFs) consisting of a very ultrathin structure with a thickness of ∼10 nm further offer a low overpotential of 260 mV at 10 mA cm −2 . In other reports, Zhang et al. 433 successfully performed the OER process with ultrathin 2D-MOF sheets prepared via electrochemical and chemical exfoliation strategies.
Recent work on the catalytic activity of 2D-MOFs has also been reported in relation to the ORR and CO 2 RR because of their layered crystal structures and high-volume modifiable porous structures. For example, Dincă et al. 434 demonstrated that ultrathin layered conductive sheets of the 2D-MOF Ni 3 (HITP) 2 (HITP = 2,3,6,7,10,11-hexaiminotriphenylene) could actively be utilized as a catalyst in an alkaline medium for the ORR process. These 2D-MOF sheets show high stability while retaining 88% of the initial current density over 8 h at 0.77 V vs. RHE. In another report, through fabricating Co x Zn 2− x (bim) 4 2D-sheets as precursors, Zhao et al. 435 successfully synthesized cobalt nanodots (Co-NDs) with bimetallic Co x Zn 2− x (bim) 4 nanosheets encapsulating few-layer graphene (Co@FLG). For the CO 2 RR, a cobalt–porphyrin-containing 2D-MOF was achieved for the selective electrochemical reduction of CO 2 to CO with enhanced stability by Peidong Yang and co-workers. 436 The results further proved that these thin-film catalysts have the highest selectivity for CO ( i.e. , 76%) at −0.7 V vs. RHE with the little-to-no substantial decrease in activity over 7 h at −0.7 V vs. RHE, and 16 mL of CO was produced. Besides, like many other porous materials, 2D-MOFs were also shown to be a supporting platform for catalytic nanoparticles because of their high specific surface areas and favorable porosity distributions. To this end, an example can be noted from Wang et al. 437 reporting that fine porous MOF-5 nanosheets can be utilized to immobilize Pd nanoparticles.
5.4. Metal-based nanostructured materials
As discussed, catalysis is one of the main uses of metal-based nanostructured materials. A continuous increase in the demand for energy, the rapid depletion of conventional energy reservoirs, and rising concerns over the emission of CO 2 have increased the challenges and urgency in the energy field. 460 Metal-based nanostructured materials are extensively being explored to produce alternative clean and renewable energy sources. A range of metal-based nanomaterials has been evaluated and is under consideration for developing robust electrodes that can be effectively applied to water splitting, batteries, and solar cells.
High energy demands have led to more pressure to improve the performances of existing highly demanded lithium-ion batteries. Researchers have focused on improving their lifetimes, sizes, and safety. 462 Nanostructured metal-oxide-based materials are promising electrode materials for use in high-performance charge-storage devices. A metal-based nanostructured electrode is evaluated as both the anode and cathode to overcome the challenges of conventional electrodes. 463 In a conventional LIB, LiCoO 2 was used as the cathode material. Controlled morphology plays a crucial role in determining the performance of a material. Powder composed of spherical particles of LiNi 0.8 Co 0.2 O 2 showed a higher tap density compared to irregular particles and the material substantially improved the power density of secondary lithium batteries. 464 Hierarchical nanostructures of metal-based oxides (such as 3D hierarchical ZnCo 2 O 4 nanostructures) have emerged as a new trend for the development of high-capacity electrodes for lithium-ion batteries. 465 Since their commercialization by Sony in the early 1990s, LIBs have achieved tremendous success in bringing portable electronic devices to the market. However, their sustainable development on the grid-scale is hampered due to limited Li resources in nature, and this is causing a continuous increase in cost. 466 Sodium-ion batteries are in the spotlight to replace powerful lithium-ion batteries due to the widespread availability of sodium and its lower cost compared with lithium. 467 It is essential to note that, in terms of energy densities for SIBs, it is difficult to bypass LIBs because of the low standard electrochemical potential and higher weight of Na. SIBs could be proved to be ideal for those applications where cost is a critical factor compared to energy density. 466
SIBs also operate similarly to LIBs, based on an intercalation mechanism. SIBs also consist of cathode and anode electrodes separated through an electrolyte. During the charging process, sodium ions are extracted from the cathode and inserted into the anode via the electrolyte. In the discharging process, the electrons leave the anode through an external circuit to reach the cathode, providing electricity to the load, whereas Na + moves to the cathode during this process. The radius of Na + (1.02 Å) is greater than that of Li + (0.76 Å), making it challenging to intercalate into electrode materials. 468 Thus, appropriate electrode materials are required in which fast Na-ion insertion and extraction is possible. However, SIBs are suffering from a lack of appropriate electrode materials. It is important to develop electrode materials that have enough interstitial space within their crystallographic structures and better electrochemical performance. Among the various proposed electrode materials, Na x MO 2 layered transition-metal oxides (M = V, Fe, Cu, Co, Ni, Cr, Mn, and their combinations) are considered to be promising electrode materials for SIBs. Layered metal oxides are considered to be promising electrode materials due to their facile scalable synthesis, simple structures, appropriate operating potentials, and high capacities. 469,470 Large volume expansion and poor kinetics during the charge–discharge process can severely affect the cyclability and performance of SIBs. One of the effective strategies to deal with the mechanical stress triggered by large volume changes is the design of hollow or porous structures. In response, three-dimensional network-based Sb 2 O 3 @Sb composite anode materials can help to relieve the volume-change-related stress through their uniform porous networks and provide better transportation channels for Na + . 471
The large volume expansion of electrodes can also be buffered via designing 2D metal-oxide materials with large interlayer spacing. The ultrathin nanosheets provide high reversible capacity with enhanced cycling stability and contribute to providing reaction sites for electrons/ions, decreasing the diffusion distance, providing effective diffusion channels, and facilitating fast charge/discharge for sodium and lithium. 2D SnO nanosheet anodes were evaluated for SIBs. The capacity and cyclic stability improved, as the number of atomic SnO layers is decreased in the sheets. 472 Sb is a promising anode material, but during the sodiation/desodiation processes, huge volume expansion of 390% is observed, which hinders its practical use. Nanostructured Sb in the form of nanorod arrays with large interval spacing displays the great capacity to accommodate volume changes during cycling. 473 A comparison of various nanostructured metal-based electrodes for various charge storage purposes is shown in Table 3 . Overall, well-structured metal or metal-based oxide nanomaterials have the capacity to resolve current issues relating to charge storage devices.
Recently, an immense focus of research has been to produce H 2 fuel via water-splitting to replace conventional fossil fuels. This will help to eliminate emissions from the use of carbonaceous species. 484 Electrochemical method are considered simple water splitting approaches, as these methods only require an applied voltage and water as inputs to produce hydrogen fuel. 485 The coupling of solar irradiation to electrochemical water splitting has enhanced the performance and reduced the process cost. Due to these reasons, this has become a hot area of research. 486 During water electrolysis, H 2 is produced through the hydrogen evolution reaction at the cathode and O 2 is produced through the oxygen evolution reaction at the anode. However, water splitting is not so straightforward, and it requires an efficient catalyst that can facilitate the splitting of water. Metal- and metal-oxide-based catalysts are extensively being explored for water splitting. For the HER reaction, Pt-based catalysts are found to be suitable, whereas for OER reactions, Ir-/Ru-based compounds are found to be benchmark catalysts. Scarcity and high cost have limited the widespread use of these metals. The barrier of noble-metal cost can be mitigated through developing noble-metal nanostructured surfaces that produce more active sites or via depositing monolayers of noble metals on low-cost materials. The alloying of noble metals with other metals has enhanced site-specific activity. 484 At present, more focus is being placed on developing noble-metal-free catalysts for water splitting. 485 Usually, an efficient electrocatalyst is characterized by: 487 a low overpotential; high stability; low production costs; and high electrocatalytic activity.
The nano-structuring of catalysts is an effective tool to boost their surface areas. The electrolysis of water occurs at the surface of a catalyst, and nanostructured catalysts provide more active sites and the better diffusion of ions and electrolytes. 484 Non-noble metals that are under observation for the development of HER electrocatalysts include nickel (Ni), tungsten (W), iron (Fe), molybdenum (Mo), cobalt (Co), and copper (Cu). 487 For instance, a noble metal-free catalyst, carbon-decorated Co 3 O 4 nanoarrays on carbon paper, required a small overpotential of 370 mV to reach a current density of 10 mA cm −2 . It can maintain a current density of 100 mA cm −2 for 413.8 h and 86.8 h under alkaline and acidic conditions, respectively. 488
Metal-based semiconductor materials play a crucial role in a range of applications. For photoelectrochemical water splitting, the semiconductor material plays a central role in the solar-to-hydrogen conversion efficiency. Some critical features are prerequisites when it comes to selecting the right semiconductor material for the photoelectrochemical splitting of water: 489 an extraordinary capacity to absorb visible light; an appropriate bandgap; suitable valence and conduction band positions; commercial feasibility; and chemical stability.
For an ideal semiconductor for water splitting, the valence band and conduction band edge positions must straddle the oxidation and the reduction potentials of water. Metal oxides have received significant attention among semiconductors due to their wide band gap distributions, remarkable photo-electrochemical stabilities, and favorable band edge positions. 490 Semiconductor-based photoelectrodes become excited upon light irradiation, and electrons from the valence band move to the unoccupied conduction band. Some of the generated electrons at the cathode surface reduce protons to hydrogen gas, whereas holes at the photoanode produce oxygen gas via water splitting. 490 As a result, various nanostructured metal oxides can be used as photoelectrode materials, such as WO 3 , 491 Cu 2 O, 492 TiO 2 , 493 ZnO, 494 SnO 2 , 495 BiVO 4 , 496 and α-Fe 2 O 3 , 490 for the efficient splitting of water. As discussed, the nano-structuring of semiconductors can significantly impact the electrode photoelectrochemical performance during water splitting.
Metal-based nanomaterials have been used for the development of sensitive sensors. These metal-based sensors can replace the complex and expensive instruments that are conventionally used for the sensing of analytes. Metal-oxide-based sensors have the interesting characteristics of low detection limits, low cost, high sensitivity, and facile operation. 497 Mostly, semiconducting metal-oxide-based sensors are used for the sensing of toxic, flammable, and exhaust gases. Semiconductor metal oxides with a size in the range of 1–100 nm have been significantly investigated as gas sensors due to their size-dependent properties. The geometry and size of a nanomaterial can considerably affect the hole and electron movement in semiconductors. 498 The surface-to-volume ratio and surface area are substantially enhanced at the nanoscale level, and this is amazingly beneficial for sensing. Chemiresistive semiconducting metal oxides are potential candidates for gas sensing due to the following features: 499 rapid response times; fast recovery times; low cost; simple electronic interfaces; user-friendliness and low maintenance; and abilities to sense a wide range of gases.
Electrode materials decorated with metal- or metal-oxide-based nanostructured materials have shown better responses and selectivity for determining various analytes over conventional electrode materials. The nano-sized metal structures act as an electrocatalyst and electronic wires to provide rapid electron transfer between the transducers and analyte molecules. 500 The electrochemical redox reaction of H 2 O 2 can be improved via the thermally controlled anchoring of Pt NPs on the electrode surface. 501
Currently, researchers are not just concentrating on the development of randomly shaped nanomaterials; instead, they are very focused on and interested in the rational design of materials with controlled nano-architectures for boosting their performances for specific applications. As a result, extensive research has been carried out to develop metal-based materials with controlled dimensions to achieve better catalytic responses. Particle morphology is a crucial factor in the performance of nanomaterials for specific applications. Laifa Shen et al. rationally designed an electrode architecture via growing mesoporous NiCo 2 O 4 nanowire arrays on carbon textiles, which boosted the electrode performance ( Fig. 37 ). 474
The same materials with different morphologies can produce different outcomes. For instance, MnO 2 nanoflowers have provided high initial sodium-ion storage capacity compared with MnO 2 nanorods. 481 Radha Narayanan and Mostafa A. El-Sayed have analyzed various nanoscale morphologies of Pt, such as tetrahedral, cubic, and near-spherical nanoparticles. The highest rate constant is observed with tetrahedral nanoparticles and the lowest rate constant was observed with cubic nanoparticles, whereas spherical nanoparticles exhibited an intermediate rate constant during catalysis. 502 Xiaowei Xie et al. found that Co 3 O 4 nanorods show high activity compared to conventional Co 3 O 4 nanoparticles for the low-temperature oxidation of CO. 503 The catalytic activity of metal-based nanomaterials is strongly affected by their shape. 504 Shape-defined mesoporous materials (TiO 2 ) have shown superior photoanode activities ( Fig. 38 ). 505 As a result, in the literature, several nanostructured morphologies of metal-based materials, such as nanotubes, 506,507 nanorods, 508,509 nanoflowers, 510 nanosheets, 511 nanowires, 512 nanocubes, 513 nanospheres, 514,515 nanocages, 516 and nanoboxes, 517 have been reported for a range of applications.
Hollow nanostructures have surfaced as an amazing class of nanostructured material, and they have received significant attention from researchers. Hollow nanostructures have the unique features of: 518,519 low density; abundant inner void spaces; large surface areas; and the ability to act as nanoscale containers with high loading capacity, nanoreactors, and nanocarriers.
Various metal-based hollow nanostructures, such as hollow SnO 2 , 520 hollow palladium nanocrystals, 521 Co–Mn mixed oxide double-shell hollow spheres, 521 hollow Cu 2 O nanocages, 522 three-dimensional hollow SnO 2 @TiO 2 spheres, 523 hollow ZnO/Co 3 O 4 nano-heterostructure, 524 triple-shell hollow α-Fe 2 O 3 , 525 and hierarchical hollow Mn-doped Ni(OH) 2 nanostructures, 526 have been developed for various applications. The presence of nanoscale hollow interiors and functional shells imparts them with great potential for gas sensing, catalysis, biomedicine, energy storage, and conversion. 519
From this discussion, it can be concluded that metal-based nanostructured materials have great potential compared to their bulk counterparts. The conversion of materials to the nanoscale is not enough to achieve high performance with better selectivity. Now, research is switching from conventional nanomaterials to more advanced and smartly designed nanomaterials. In modern research, nanomaterials are being designed with better-controlled morphologies and regulated features.
5.5. Core–shell nanoparticles
A spherical nanoparticle core–shell nanostructure is a practical way to introduce multiple functionalities on the nanoscopic length scale. 528 The properties arising from the core or shell can be different, and these properties can be tuned via controlling the ratio of the constituent materials. The shape, size, and composition play a critical role in tuning the core–shell nanoparticle properties. 529 The shell material can help to improve the chemical and thermal stabilities of the core material. The core–shell design has become effective where an inexpensive material cannot be used directly due to its instability or easily oxidizable nature. The core can consist of an easily oxidizable inexpensive metal, whereas the shell might consist of noble metals, oxides, polymers, or silica. 530 For instance, magnetic nanoparticles when prepared can be sensitive toward air, acids, and bases. Magnetic nanoparticles can be protected via coating with organic or inorganic shells. 528
Core–shell metal nanoparticles are an emerging nanostructured material with great potential in the fields of energy and catalysis. 531 The first report of core–shell nanoparticles (2007) for supercapacitor applications consisted of a polyaniline/multi-walled-carbon-nanotube composite (PANI/MWNTs). 532 Metal-based core–shell structured nanoparticles have shown enhanced catalytic performance due to their shape-controlled properties. 533 Ming-Yu Kuo et al. developed Au@Cu 2 O core–shell particles with controllable shell thicknesses that acted as a dual-functional catalyst. The shell thickness of Cu 2 O increased with an increasing concentration of Cu 2+ precursor. The thicknesses of the shells of Au@Cu 2 O-1.5 (12.2 ± 1.7 nm), Au@Cu 2 O-2 (13.2 ± 1.8 nm), Au@Cu 2 O-3 (18.2 ± 2.2 nm), and Au@Cu 2 O-4 (20.8 ± 2.5 nm) due to various concentrations are shown in Fig. 40 . 534 A NiO@SiO 2 core–shell catalyst provided a higher yield of acrylic acid from acetylene hydroxycarbonylation. 535 Core–shell architecture can be used to prevent active metal nanoparticles from oxidation during operation. For instance, a plasmonic photocatalyst was developed that consisted of silver nanoparticles embedded in titanium dioxide. The direct contact of Ag with TiO 2 could lead to its oxidization; this is prevented via developing core–shell architecture in which Ag is used as the core and SiO 2 is used as a shell to protect it. 536 Another excellent option is to replace an expensive core with a non-noble metal to reduce the core–shell cost while using a thin layer of a noble metal that consumes a small amount of metal as the shell. This will ensure the prolonged stability of the catalyst during operation. 533 Overall, core–shell morphologies provide better catalytic activity due to the synergistic effect of the metallic core–shell components. 152
Among the several classes of nanomaterials, core–shell nanoparticles are found to be more promising for different biomedical applications. For instance, magnetic nanoparticles are considered to be useful for biomedical applications due to the following reasons: (a) aggregation is prevented due to superparamagnetism; (b) delivery and separation can be controlled using an external magnetic field; (c) they can be appropriately dispersed; and (d) there is the possibility of functionalization. A range of magnetic nanoparticles is available, such as NiO, Ni, Co, and Mn 3 O 4 . The most famous example is iron oxide, but uncoated iron oxides are unstable under physiological conditions. This may result in controlled drug delivery failure due to improper ligand surface binding and the promotion of the formation of harmful free radicals. Therefore, the formation of shells around magnetic nanoparticles has tremendous significance for biomedical applications. 537 One of the approaches is to use gold shells on magnetic nanoparticles. Au NPs are also called surface plasmons and they substantially enhanced the absorption of light in the visible and near-infrared regions. Thus, coating magnetic nanoparticles with a Au shell can result in a core–shell nanostructure that displays both optical and magnetic functionality in combination. 529
Numerous biocompatible core–shell nanoparticles are being developed for photothermal therapy, as core–shell materials are found to be useful for photothermal therapy. Hui Wang et al. have developed bifunctional core–shell nanoparticles for dual-modal imaging-guided photothermal therapy. The core–shell nanoparticles consist of a magnetic ∼9.1 nm core of Fe 3 O 4 covered by an approximately 3.4 nm fluorescent carbon shell. The Fe 3 O 4 core leads to superparamagnetic behavior, whereas the carbon shell provides near-infrared (NIR) fluorescence properties. The bifunctional nanoparticles have shown dual-modal imaging capacity both in vivo and in vitro . The iron oxide–carbon core–shell nanoparticles absorbed and converted near-infrared light to heat, facilitating photothermal therapy. 538 Au-Based core–shell structures are also being prepared for photothermal therapy. Bulk gold is biocompatible, but Au NPs can accumulate in the spleen and liver, causing severe toxicity. Koo Chul Kwon et al. have developed Au-NP-based core–shell structures that did not result in any gross or histological lesions in the major organs of mice, which revealed that this is a potent and safe agent for photothermal cancer therapy. The core–shell nanoparticles consisted of proteinticle/gold (PGCS-NP) and were developed via proteinticle surface engineering. PGCS-NP was injected intravenously into mice with tumors, and the injected core–shell nanoparticles successfully reached the EGFR-expressing tumor cells. The tumor size was significantly reduced upon exposure to near-infrared laser irradiation ( Fig. 41 ). No accumulation of Au NPs was observed in the mice organs, which indicated that PGCS-NP disassembled into many tiny gold dots, which were easily excreted by the kidneys and liver without causing any toxicity. 539 In another example, multifunctional Au@graphene oxide nanocolloid core@shell nanoparticles were developed, in which the core and shell consisted of gold and a graphene oxide nanocolloid, respectively. The developed core–shell structure showed multifunctional properties, allowing Raman bioimaging and photothermal/photodynamic therapy with low toxicity. 540 Apart from this, numerous other core–shell nanoparticles, such as polydopamine–mesoporous silica core–shell nanoparticles, 541 AuPd@PVP core–shell nanoparticles, 542 Au@Cu 2− x S core–shell nanoparticles, 543 bismuth sulfide@mesoporous silica core–shell nanoparticles, 544 and Ag@S-nitrosothiol core–shell nanoparticles, have been used for photothermal therapy. 545
Due to their unique features and the combination of properties from the shell and core, these core–shell nanoparticles have received considerable interest in many fields, ranging from materials chemistry to the biomedical field. For electrochemical reactions, the core–shell structure conductivity can be enhanced via conducting polymers, carbon materials, and metals. Core–shell nanoparticles as electrode materials showed better performance compared to single components. Most of the core materials are prepared via hydrothermal methods, and shells can be prepared via hydrothermal or electrodeposition methods. 546 Even though significant progress has been made relating to the synthesis methods of core–shell materials, a major challenge is the high-quality production of core–shell materials in more effective ways for required applications, specifically biomedical applications.
6. Challenges and future perspectives
(a) The presence of defects in nanomaterials can affect their performance and their inherent characteristics can be compromised. For instance, carbon nanotubes are one of the strongest materials that are known. However, impurities, discontinuous tube lengths, defects, and random orientations can substantially impair the tensile strength of carbon nanotubes. 547
(b) The synthesis of nanomaterials through cost-effective routes is another major challenge. High-quality nanomaterials are generally produced using sophisticated instrumentation and harsh conditions, limiting their large-scale production. This issue is more critical for the synthesis of 2D nanomaterials. Most of the methods that have been adopted for large-scale production are low cost, and these methods generally produce materials with defects that are of poor quality. The controlled synthesis of nanomaterials is still a challenging job. For example, a crucial challenge associated with carbon nanotube synthesis is to achieve chiral selectivity, conductivity, and precisely controlled diameters. 548,549 Obtaining structurally pure nanomaterials is the only way to achieve the theoretically calculated characteristics described in the literature. More focused efforts are required to develop new synthesis methods that overcome the challenges associated with conventional methods.
(c) The agglomeration of particles at the nanoscale level is an inherent issue that substantially damages performance in relevant fields. Most nanomaterials start to agglomerate when they encounter each other. The process of agglomeration may be due to physical entanglement, electrostatic interactions, or high surface energy. 550 CNTs undergo van der Waals interactions and form bundles, making it difficult to align or properly disperse them in polymer matrices. 159 Similarly, graphene agglomeration is triggered by the basal planes of graphene sheets due to π–π interactions and van der Waals forces. Due to severe agglomeration, the high surface areas and other unique graphene features are compromised. These challenges hinder the practical application of high-throughput electrode materials or composite materials for various applications. 551
(d) The efficiency of nanomaterials can be tuned via developing 3D architectures. 3D architectures have been tried with several nanomaterials, such as graphene, to improve their inherent features. 3D architectures of 2D graphene have provided high specific surface areas and fast mass and electron transport kinetics. This has become possible due to the combination of the exceptional intrinsic properties of graphene and 3D porous structures. 194,552 The combination of graphene and CNT assemblies into 3-D architectures has emerged as the most investigated nanotechnology research area. Porous architectures of other nanomaterials can be developed to enhance their catalysis performance through providing nanomaterial interior availability.
(e) 2D ultrathin materials are an outstanding class of nanomaterial with promising theoretical properties; however, very little experimental evaluation of these materials has been done, apart from the case of graphene. The synthesis and stability of 2D ultrathin materials are some of the major challenges associated with them. In the future, more focus is anticipated to be placed on their synthesis and practical utilization.
(f) Nanomaterial utilization in industry is being increased, and there is also demand for nanoscale material production at higher rates. Moreover, nanotechnology research has vast horizons; the exploration of new nanomaterials with fascinating features will continue and, in the future, more areas will be discovered. One of the significant concerns relating to nanomaterials that cannot be overlooked is their toxicity, which is still poorly understood, and this is a serious concern relating to their environmental, domestic, and industrial use. The extent to which nanoparticle-based materials can contribute to cellular toxicity is unclear. 553 There is a need for the scientific community to put efforts into reducing the knowledge gap between the rapid development of nanomaterials and their possible in vivo toxicity. A proper and systematic understanding of the interaction of nanomaterials with cells, tissues, and proteins is critical for the safe design and commercialization of nanotechnology. 14
The future of advanced technology is linked with advancements in the field of nanotechnology. The dream of clean energy production is becoming possible with the advancement of nanomaterial-based engineering strategies. These materials have shown promising results, leading to new generations of hydrogen fuel cells and solar cells, acting as efficient catalysts for water splitting, and showing excellent capacity for hydrogen storage. Nanomaterials have a great future in the field of nanomedicine. Nanocarriers can be used for the delivery of therapeutic molecules.
7. Conclusions
Conflicts of interest, acknowledgements.
- F. J. Heiligtag and M. Niederberger, Mater. Today , 2013, 16 , 262–271 CrossRef CAS .
- P. Walter, E. Welcomme, P. Hallégot, N. J. Zaluzec, C. Deeb, J. Castaing, P. Veyssière, R. Bréniaux, J.-L. Lévêque and G. Tsoucaris, Nano Lett. , 2006, 6 , 2215–2219 CrossRef CAS PubMed .
- J. Jeevanandam, A. Barhoum, Y. S. Chan, A. Dufresne and M. K. Danquah, Beilstein J. Nanotechnol. , 2018, 9 , 1050–1074 CrossRef CAS PubMed .
- I. Freestone, N. Meeks, M. Sax and C. Higgitt, Gold Bull. , 2007, 40 , 270–277 CrossRef CAS .
- A. Santamaria, Nanotoxicity , Humana Press, Totowa, NJ, 2012, pp. 1–12 Search PubMed .
- R. P. Feynman, Eng. Sci. , 1960, 23 , 22–36 Search PubMed .
- S. Bayda, M. Adeel, T. Tuccinardi, M. Cordani and F. Rizzolio, Molecules , 2019, 25 , 112 CrossRef PubMed .
- M. Nasrollahzadeh, S. M. Sajadi, M. Sajjadi and Z. Issaabadi, Interface Science and Technology , Elsevier B.V., 2019, vol. 28, pp. 1–27 Search PubMed .
- N. Taniguchi, Proc. Int. Conf. Prod. Eng. Issue PART II , 1974, 18–23.
- G. Binnig and H. Rohrer, US Pat. , US4343993A, 1982 Search PubMed .
- G. Binnig, C. F. Quate and C. Gerber, Phys. Rev. Lett. , 1986, 56 , 930–933 CrossRef PubMed .
- H.-J. Butt, B. Cappella and M. Kappl, Surf. Sci. Rep. , 2005, 59 , 1–152 CrossRef CAS .
- A. C. Marques, M. Vale, D. Vicente, M. Schreck, E. Tervoort and M. Niederberger, Global Challenges , 2021, 2000116 CrossRef PubMed .
- S. Sharifi, S. Behzadi, S. Laurent, M. Laird Forrest, P. Stroeve and M. Mahmoudi, Chem. Soc. Rev. , 2012, 41 , 2323–2343 RSC .
- Nat. Nanotechnol. , 2019, 14 , 193 Search PubMed .
- P. N. Sudha, K. Sangeetha, K. Vijayalakshmi and A. Barhoum, Emerging Applications of Nanoparticles and Architecture Nanostructures , Elsevier, 2018, pp. 341–384 Search PubMed .
- W. G. Kreyling, M. Semmler-Behnke and Q. Chaudhry, Nano Today , 2010, 5 , 165–168 CrossRef .
- B. Bhushan, Springer Handbooks , Springer, 2017, pp. 1–19 Search PubMed .
- F. Trotta and A. Mele, Nanosponges: From Fundamentals to Applications , 2019, pp. 1–24 Search PubMed .
- Nanoanalytics , ed. S. Shtykov, De Gruyter, Berlin, Boston, 2018 Search PubMed .
- C. J. Murphy and N. R. Jana, Adv. Mater. , 2002, 14 , 80–82 CrossRef CAS .
- P. Moriarty, Rep. Prog. Phys. , 2001, 64 , 297–381 CrossRef CAS .
- B. Chen, J. R. G. Evans, H. C. Greenwell, P. Boulet, P. V. Coveney, A. A. Bowden and A. Whiting, Chem. Soc. Rev. , 2008, 37 , 568–594 RSC .
- P. Khanna, A. Kaur and D. Goyal, J. Microbiol. Methods , 2019, 163 , 105656 CrossRef CAS PubMed .
- S. Zhuang, E. S. Lee, L. Lei, B. B. Nunna, L. Kuang and W. Zhang, Int. J. Energy Res. , 2016, 40 , 2136–2149 CrossRef CAS .
- T. Prasad Yadav, R. Manohar Yadav and D. Pratap Singh, Nanosci. Nanotechnol. , 2012, 2 , 22–48 CrossRef .
- H. Lyu, B. Gao, F. He, C. Ding, J. Tang and J. C. Crittenden, ACS Sustainable Chem. Eng. , 2017, 5 , 9568–9585 CrossRef CAS .
- R. Ostermann, J. Cravillon, C. Weidmann, M. Wiebcke and B. M. Smarsly, Chem. Commun. , 2011, 47 , 442–444 RSC .
- P. S. Kumar, J. Sundaramurthy, S. Sundarrajan, V. J. Babu, G. Singh, S. I. Allakhverdiev and S. Ramakrishna, Energy Environ. Sci. , 2014, 7 , 3192–3222 RSC .
- P. Du, L. Song, J. Xiong, N. Li, Z. Xi, L. Wang, D. Jin, S. Guo and Y. Yuan, Electrochim. Acta , 2012, 78 , 392–397 CrossRef CAS .
- A. Pimpin and W. Srituravanich, Eng. J. , 2012, 16 , 37–56 CrossRef .
- Z. Szabó, J. Volk, E. Fülöp, A. Deák and I. Bársony, Photonics Nanostruct. , 2013, 11 , 1–7 CrossRef .
- C.-W. Kuo, J.-Y. Shiu, Y.-H. Cho and P. Chen, Adv. Mater. , 2003, 15 , 1065–1068 CrossRef CAS .
- Y. Yin, B. Gates and Y. Xia, Adv. Mater. , 2000, 12 , 1426–1430 CrossRef CAS .
- K. Xu and J. Chen, Appl. Nanosci. , 2020, 10 , 1013–1022 CrossRef CAS .
- R. Matsumoto, S. Adachi, E. H. S. Sadki, S. Yamamoto, H. Tanaka, H. Takeya and Y. Takano, ACS Appl. Electron. Mater. , 2020, 2 , 677–682 CrossRef CAS .
- V. Garg, R. G. Mote and J. Fu, Appl. Surf. Sci. , 2020, 526 , 146644 CrossRef CAS .
- P. Ayyub, R. Chandra, P. Taneja, A. K. Sharma and R. Pinto, Appl. Phys. A: Mater. Sci. Process. , 2001, 73 , 67–73 CrossRef CAS .
- H. H. Son, G. H. Seo, U. Jeong, D. Y. Shin and S. J. Kim, Int. J. Heat Mass Transfer , 2017, 113 , 115–128 CrossRef CAS .
- H. Wender, P. Migowski, A. F. Feil, S. R. Teixeira and J. Dupont, Coord. Chem. Rev. , 2013, 257 , 2468–2483 CrossRef CAS .
- J. Muñoz-García, L. Vázquez, R. Cuerno, J. A. Sánchez-García, M. Castro and R. Gago, Toward Functional Nanomaterials , Springer US, New York, NY, 2009, pp. 323–398 Search PubMed .
- J. H. Nam, M. J. Jang, H. Y. Jang, W. Park, X. Wang, S. M. Choi and B. Cho, J. Energy Chem. , 2020, 47 , 107–111 CrossRef .
- M. Nie, K. Sun and D. D. Meng, J. Appl. Phys. , 2009, 106 , 054314 CrossRef .
- D. Zhang, K. Ye, Y. Yao, F. Liang, T. Qu, W. Ma, B. Yang, Y. Dai and T. Watanabe, Carbon , 2019, 142 , 278–284 CrossRef CAS .
- C. M. Lieber and C.-C. Chen, Solid State Physics – Advances in Research and Applications , Academic Press, 1994, vol. 48, pp. 109–148 Search PubMed .
- J. M. Jones, R. P. Malcolm, K. M. Thomas and S. H. Botrell, Carbon , 1996, 34 , 231–237 CrossRef CAS .
- F. Liang, M. Tanaka, S. Choi and T. Watanabe, Carbon , 2017, 117 , 100–111 CrossRef CAS .
- F. Liang, T. Shimizu, M. Tanaka, S. Choi and T. Watanabe, Diamond Relat. Mater. , 2012, 30 , 70–76 CrossRef CAS .
- N. Li, Z. Wang, K. Zhao, Z. Shi, Z. Gu and S. Xu, Carbon , 2010, 48 , 1580–1585 CrossRef CAS .
- Z.-S. Wu, W. Ren, L. Gao, J. Zhao, Z. Chen, B. Liu, D. Tang, B. Yu, C. Jiang and H.-M. Cheng, ACS Nano , 2009, 3 , 411–417 CrossRef CAS PubMed .
- V. Amendola and M. Meneghetti, Phys. Chem. Chem. Phys. , 2009, 11 , 3805 RSC .
- J. Zhang, M. Chaker and D. Ma, J. Colloid Interface Sci. , 2017, 489 , 138–149 CrossRef CAS PubMed .
- R. A. Ismail, M. H. Mohsin, A. K. Ali, K. I. Hassoon and S. Erten-Ela, Phys. E , 2020, 119 , 113997 CrossRef CAS .
- J. Chrzanowska, J. Hoffman, A. Małolepszy, M. Mazurkiewicz, T. A. Kowalewski, Z. Szymanski and L. Stobinski, Phys. Status Solidi , 2015, 252 , 1860–1867 CrossRef CAS .
- J. Duque, B. Madrigal, H. Riascos and Y. Avila, Colloids Interfaces , 2019, 3 , 25 CrossRef CAS .
- S. S. Su and I. Chang, Commercialization of Nanotechnologies–A Case Study Approach , Springer International Publishing, Cham, 2018, pp. 15–29 Search PubMed .
- H. Park, D. A. Reddy, Y. Kim, S. Lee, R. Ma and T. K. Kim, Chem. – A Eur. J. , 2017, 23 , 13112–13119 CrossRef CAS PubMed .
- A. C. Jones and M. L. Hitchman, in Chemical Vapour Deposition , ed. A. C. Jones and M. L. Hitchman, Royal Society of Chemistry, Cambridge, 2008, pp. 1–36 Search PubMed .
- K. A. Shah and B. A. Tali, Mater. Sci. Semicond. Process. , 2016, 41 , 67–82 CrossRef CAS .
- H. Ago, Frontiers of Graphene and Carbon Nanotubes , Springer, Japan, Tokyo, 2015, pp. 3–20 Search PubMed .
- P. Machac, S. Cichon, L. Lapcak and L. Fekete, Graphene Technol. , 2020, 5 , 9–17 CrossRef .
- Q. Wu, W. Wongwiriyapan, J.-H. Park, S. Park, S. J. Jung, T. Jeong, S. Lee, Y. H. Lee and Y. J. Song, Curr. Appl. Phys. , 2016, 16 , 1175–1191 CrossRef .
- X. Wu, G. Q. (Max) Lu and L. Wang, Energy Environ. Sci. , 2011, 4 , 3565 RSC .
- S. Cao, C. Zhao, T. Han and L. Peng, Mater. Lett. , 2016, 169 , 17–20 CrossRef CAS .
- J. Li, Q. Wu and J. Wu, Handbook of Nanoparticles , Springer International Publishing, Cham, 2015, pp. 1–28 Search PubMed .
- A. Chen and P. Holt-Hindle, Chem. Rev. , 2010, 110 , 3767–3804 CrossRef CAS .
- L.-Y. Meng, B. Wang, M.-G. Ma and K.-L. Lin, Mater. Today Chem. , 2016, 1–2 , 63–83 CrossRef .
- Y. Dong, X. Du, P. Liang and X. Man, Inorg. Chem. Commun. , 2020, 115 , 107883 CrossRef CAS .
- Y. Jiang, Z. Peng, S. Zhang, F. Li, Z. Liu, J. Zhang, Y. Liu and K. Wang, Ceram. Int. , 2018, 44 , 6115–6126 CrossRef CAS .
- B. Chai, M. Xu, J. Yan and Z. Ren, Appl. Surf. Sci. , 2018, 430 , 523–530 CrossRef CAS .
- A. E. Danks, S. R. Hall and Z. Schnepp, Mater. Horiz. , 2016, 3 , 91–112 RSC .
- T. K. Tseng, Y. S. Lin, Y. J. Chen and H. Chu, Int. J. Mol. Sci. , 2010, 11 , 2336–2361 CrossRef CAS .
- M. Parashar, V. K. Shukla and R. Singh, J. Mater. Sci.: Mater. Electron. , 2020, 31 , 3729–3749 CrossRef CAS .
- L. Znaidi, Mater. Sci. Eng., B , 2010, 174 , 18–30 CrossRef CAS .
- C. de Coelho Escobar and J. H. Z. dos Santos, J. Sep. Sci. , 2014, 37 , 868–875 CrossRef PubMed .
- Y. Liu, J. Goebl and Y. Yin, Chem. Soc. Rev. , 2013, 42 , 2610–2653 RSC .
- W. Li and D. Zhao, Chem. Commun. , 2013, 49 , 943–946 RSC .
- R. R. Poolakkandy and M. M. Menamparambath, Nanoscale Adv. , 2020, 2 , 5015–5045 RSC .
- C. T. Kresge, M. E. Leonowicz, W. J. Roth, J. C. Vartuli and J. S. Beck, Nature , 1992, 359 , 710–712 CrossRef CAS .
- J. S. Beck, J. C. Vartuli, W. J. Roth, M. E. Leonowicz, C. T. Kresge, K. D. Schmitt, C. T. W. Chu, D. H. Olson, E. W. Sheppard, S. B. McCullen, J. B. Higgins and J. L. Schlenker, J. Am. Chem. Soc. , 1992, 114 , 10834–10843 CrossRef CAS .
- J. Liu, T. Yang, D.-W. Wang, G. Q. Lu, D. Zhao and S. Z. Qiao, Nat. Commun. , 2013, 4 , 2798 CrossRef .
- R. Lv, C. Cao, H. Zhai, D. Wang, S. Liu and H. Zhu, Solid State Commun. , 2004, 130 , 241–245 CrossRef .
- L. Martins, M. A. Alves Rosa, S. H. Pulcinelli and C. V. Santilli, Microporous Mesoporous Mater. , 2010, 132 , 268–275 CrossRef CAS .
- T. Tang, T. Zhang, W. Li, X. Huang, X. Wang, H. Qiu and Y. Hou, Nanoscale , 2019, 11 , 7440–7446 RSC .
- B. Szczęśniak, J. Choma and M. Jaroniec, Chem. Commun. , 2020, 56 , 7836–7848 RSC .
- Y. Yamauchi and K. Kuroda, Chem. – Asian J. , 2008, 3 , 664–676 CrossRef CAS PubMed .
- S. J. Hurst, E. K. Payne, L. Qin and C. A. Mirkin, Angew. Chem., Int. Ed. , 2006, 45 , 2672–2692 CrossRef CAS PubMed .
- M. A. Malik, M. Y. Wani and M. A. Hashim, Arabian J. Chem. , 2012, 5 , 397–417 CrossRef CAS .
- M. Soleimani Zohr Shiri, W. Henderson and M. R. Mucalo, Materials , 2019, 12 , 1896 CrossRef PubMed .
- T.-D. Nguyen, Nanoscale , 2013, 5 , 9455 RSC .
- S. Yi, F. Dai, C. Zhao and Y. Si, Sci. Rep. , 2017, 7 , 9806 CrossRef .
- R. Jose Varghese, E. H. M. Sakho, S. Parani, S. Thomas, O. S. Oluwafemi and J. Wu, Nanomaterials for Solar Cell Applications , Elsevier, 2019, pp. 75–95 Search PubMed .
- E. Roduner, Chem. Soc. Rev. , 2006, 35 , 583 RSC .
- A. J. Mannix, X.-F. Zhou, B. Kiraly, J. D. Wood, D. Alducin, B. D. Myers, X. Liu, B. L. Fisher, U. Santiago, J. R. Guest, M. J. Yacaman, A. Ponce, A. R. Oganov, M. C. Hersam and N. P. Guisinger, Science , 2015, 350 , 1513–1516 CrossRef CAS PubMed .
- Nanobiotechnology: Concepts and Applications in Health, Agriculture, and Environment , ed. R. S. Tomar, A. Jyoti and S. Kaushik, 1st edn, 2020 Search PubMed .
- A. P. Alivisatos, Science , 1996, 271 , 933–937 CrossRef CAS .
- R. Tomar, A. A. Abdala, R. G. Chaudhary and N. B. Singh, Mater. Today: Proc. , 2020, 29 , 967–973, DOI: 10.1016/j.matpr.2020.04.144 .
- L. D. Geoffrion and G. Guisbiers, J. Phys. Chem. Solids , 2020, 140 , 109320 CrossRef CAS .
- S. K. Krishnan, E. Singh, P. Singh, M. Meyyappan and H. S. Nalwa, RSC Adv. , 2019, 9 , 8778–8881 RSC .
- Q. Wu, W. Miao, Y. Zhang, H. Gao and D. Hui, Nanotechnol. Rev. , 2020, 9 , 259–273 CrossRef CAS .
- W. Zhu, Y. Guo, B. Ma, X. Yang, Y. Li, P. Li, Y. Zhou and M. Shuai, Int. J. Hydrogen Energy , 2020, 45 , 8385–8395 CrossRef CAS .
- X. Liu, M. Xu, L. Wan, H. Zhu, K. Yao, R. Linguerri, G. Chambaud, Y. Han and C. Meng, ACS Catal. , 2020, 10 , 3084–3093 CrossRef CAS .
- P. Makvandi, C. Wang, E. N. Zare, A. Borzacchiello, L. Niu and F. R. Tay, Adv. Funct. Mater. , 2020, 30 , 1910021 CrossRef CAS .
- E. Castro, A. H. Garcia, G. Zavala and L. Echegoyen, J. Mater. Chem. B , 2017, 5 , 6523–6535 RSC .
- M. S. Mauter and M. Elimelech, Environ. Sci. Technol. , 2008, 42 , 5843–5859 CrossRef CAS PubMed .
- L. Chen, S. Zhao, Q. Hasi, X. Luo, C. Zhang, H. Li and A. Li, Global Challenges , 2020, 4 , 1900098 CrossRef PubMed .
- R. G. Mendes, A. Bachmatiuk, B. Büchner, G. Cuniberti and M. H. Rümmeli, J. Mater. Chem. B , 2013, 1 , 401–428 RSC .
- M. T. Beck and G. Mándi, Fullerene Sci. Technol. , 1997, 5 , 291–310 CrossRef CAS .
- X. Fan, N. Soin, H. Li, H. Li, X. Xia and J. Geng, Energy Environ. Mater. , 2020, 3 , 469–491, DOI: 10.1002/eem2.12071 .
- S. Goodarzi, T. Da Ros, J. Conde, F. Sefat and M. Mozafari, Mater. Today , 2017, 20 , 460–480 CrossRef CAS .
- P. Ehrenfreund and B. H. Foing, Science , 2010, 329 , 1159–1160 CrossRef CAS PubMed .
- J. C. Withers, R. O. Loutfy and T. P. Lowe, Fullerene Sci. Technol. , 1997, 5 , 1–31 CrossRef .
- A. Rodríguez-Fortea, A. L. Balch and J. M. Poblet, Chem. Soc. Rev. , 2011, 40 , 3551 RSC .
- N. A. Kaskhedikar and J. Maier, Adv. Mater. , 2009, 21 , 2664–2680 CrossRef CAS .
- A. A. Popov, S. Yang and L. Dunsch, Chem. Rev. , 2013, 113 , 5989–6113 CrossRef CAS .
- B. Q. Mercado, C. M. Beavers, M. M. Olmstead, M. N. Chaur, K. Walker, B. C. Holloway, L. Echegoyen and A. L. Balch, J. Am. Chem. Soc. , 2008, 130 , 7854–7855 CrossRef CAS PubMed .
- S. Yang and C.-R. Wang, Endohedral Fullerenes , World Scientific, 2014 Search PubMed .
- H. Okada, T. Komuro, T. Sakai, Y. Matsuo, Y. Ono, K. Omote, K. Yokoo, K. Kawachi, Y. Kasama, S. Ono, R. Hatakeyama, T. Kaneko and H. Tobita, RSC Adv. , 2012, 2 , 10624 RSC .
- H. Bai, H. Gao, W. Feng, Y. Zhao and Y. Wu, Nanomaterials , 2019, 9 , 630 CrossRef CAS .
- O. V. Pupysheva, A. A. Farajian and B. I. Yakobson, Nano Lett. , 2008, 8 , 767–774 CrossRef CAS .
- M. Gaboardi, N. Sarzi Amadé, M. Aramini, C. Milanese, G. Magnani, S. Sanna, M. Riccò and D. Pontiroli, Carbon , 2017, 120 , 77–82 CrossRef CAS .
- A. Shokuhi Rad and K. Ayub, Comput. Theor. Chem. , 2017, 1121 , 68–75 CrossRef CAS .
- D. Guldi and N. Martin, Comprehensive Nanoscience and Technology , Elsevier, 2011, pp. 379–398 Search PubMed .
- L. Sánchez, N. Martín and D. M. Guldi, Angew. Chem., Int. Ed. , 2005, 44 , 5374–5382 CrossRef PubMed .
- X. Zhang, Y. Ma, S. Fu and A. Zhang, Nanomaterials , 2019, 9 , 1647 CrossRef CAS PubMed .
- N. Martin and G. Bodwell, Acc. Chem. Res. , 2019, 52 , 2757–2759 CrossRef CAS PubMed .
- K. Harano and E. Nakamura, Acc. Chem. Res. , 2019, 52 , 2090–2100 CrossRef CAS PubMed .
- A. Montellano, T. Da Ros, A. Bianco and M. Prato, Nanoscale , 2011, 3 , 4035 RSC .
- E. Alipour, F. Alimohammady, A. Yumashev and A. Maseleno, J. Mol. Model. , 2020, 26 , 7 CrossRef CAS PubMed .
- M.-S. Lin, R.-T. Chen, N.-Y. Yu, L.-C. Sun, Y. Liu, C.-H. Cui, S.-Y. Xie, R.-B. Huang and L.-S. Zheng, Colloids Surf., B , 2017, 159 , 613–619 CrossRef CAS PubMed .
- K. Minami, K. Okamoto, K. Harano, E. Noiri and E. Nakamura, ACS Appl. Mater. Interfaces , 2018, 10 , 19347–19354 CrossRef CAS PubMed .
- K. Minami, K. Okamoto, K. Doi, K. Harano, E. Noiri and E. Nakamura, Sci. Rep. , 2015, 4 , 4916 CrossRef PubMed .
- S. S. Babu, H. Möhwald and T. Nakanishi, Chem. Soc. Rev. , 2010, 39 , 4021 RSC .
- N. Furuuchi, R. Shrestha, Y. Yamashita, T. Hirao, K. Ariga and L. Shrestha, Sensors , 2019, 19 , 267 CrossRef PubMed .
- J. Coro, M. Suárez, L. S. R. Silva, K. I. B. Eguiluz and G. R. Salazar-Banda, Int. J. Hydrogen Energy , 2016, 41 , 17944–17959 CrossRef CAS .
- N. Yousfi-Steiner, P. Moçotéguy, D. Candusso and D. Hissel, J. Power Sources , 2009, 194 , 130–145 CrossRef CAS .
- A. R. Tuktarov, A. A. Khuzin and U. M. Dzhemilev, Pet. Chem. , 2020, 60 , 113–133 CrossRef CAS .
- I. Jeon, A. Shawky, H.-S. Lin, S. Seo, H. Okada, J.-W. Lee, A. Pal, S. Tan, A. Anisimov, E. I. Kauppinen, Y. Yang, S. Manzhos, S. Maruyama and Y. Matsuo, J. Am. Chem. Soc. , 2019, 141 , 16553–16558 CrossRef CAS PubMed .
- Y. Takabayashi and K. Prassides, Philos. Trans. R. Soc., A , 2016, 374 , 20150320 CrossRef PubMed .
- M. A. Rafiee, F. Yavari, J. Rafiee and N. Koratkar, J. Nanopart. Res. , 2011, 13 , 733–737 CrossRef CAS .
- Y. Pan, Z. Guo, S. Ran and Z. Fang, J. Appl. Polym. Sci. , 2020, 137 , 47538 CrossRef .
- S. Iijima, Nature , 1991, 354 , 56–58 CrossRef CAS .
- S. Iijima and T. Ichihashi, Nature , 1993, 363 , 603–605 CrossRef CAS .
- I. V. Zaporotskova, N. P. Boroznina, Y. N. Parkhomenko and L. V. Kozhitov, Mod. Electron. Mater. , 2016, 2 , 95–105 CrossRef .
- X.-Q. Li, P.-X. Hou, C. Liu and H.-M. Cheng, Carbon , 2019, 147 , 187–198 CrossRef CAS .
- C. Shen, A. H. Brozena and Y. Wang, Nanoscale , 2011, 3 , 503–518 RSC .
- V. Popov, Mater. Sci. Eng., R , 2004, 43 , 61–102 CrossRef .
- Z. F. Ren, Science , 1998, 282 , 1105–1107 CrossRef CAS .
- A. G. Rinzler, J. Liu, H. Dai, P. Nikolaev, C. B. Huffman, F. J. Rodríguez-Macías, P. J. Boul, A. H. Lu, D. Heymann, D. T. Colbert, R. S. Lee, J. E. Fischer, A. M. Rao, P. C. Eklund and R. E. Smalley, Appl. Phys. A: Mater. Sci. Process. , 1998, 67 , 29–37 CrossRef CAS .
- P. Nikolaev, M. J. Bronikowski, R. K. Bradley, F. Rohmund, D. T. Colbert, K. Smith and R. E. Smalley, Chem. Phys. Lett. , 1999, 313 , 91–97 CrossRef CAS .
- N. Baig, M. Sajid and T. A. Saleh, TrAC, Trends Anal. Chem. , 2019, 111 , 47–61 CrossRef CAS .
- X.-M. Liu, Z. Dong Huang, S. Woon Oh, B. Zhang, P.-C. Ma, M. M. F. Yuen and J.-K. Kim, Compos. Sci. Technol. , 2012, 72 , 121–144 CrossRef CAS .
- T. W. Odom, J.-L. Huang, P. Kim and C. M. Lieber, Nature , 1998, 391 , 62–64 CrossRef CAS .
- K. Balasubramanian and M. Burghard, Small , 2005, 1 , 180–192 CrossRef CAS PubMed .
- J. R. Xiao, B. A. Gama and J. W. Gillespie, Int. J. Solids Struct. , 2005, 42 , 3075–3092 CrossRef .
- H. Kim, M. Wang, S. K. Lee, J. Kang, J.-D. Nam, L. Ci and J. Suhr, Sci. Rep. , 2017, 7 , 9512 CrossRef PubMed .
- J. H. Lehman, M. Terrones, E. Mansfield, K. E. Hurst and V. Meunier, Carbon , 2011, 49 , 2581–2602 CrossRef CAS .
- N. G. Sahoo, S. Rana, J. W. Cho, L. Li and S. H. Chan, Prog. Polym. Sci. , 2010, 35 , 837–867 CrossRef CAS .
- T. Kuang, L. Chang, F. Chen, Y. Sheng, D. Fu and X. Peng, Carbon , 2016, 105 , 305–313 CrossRef CAS .
- Z. Wei, M. Kondratenko, L. H. Dao and D. F. Perepichka, J. Am. Chem. Soc. , 2006, 128 , 3134–3135 CrossRef CAS PubMed .
- M. Bockrath, Science , 2001, 291 , 283–285 CrossRef CAS PubMed .
- M. Rother, M. Brohmann, S. Yang, S. B. Grimm, S. P. Schießl, A. Graf and J. Zaumseil, Adv. Electron. Mater. , 2017, 3 , 1700080 CrossRef .
- J.-M. Bonard, M. Croci, C. Klinke, R. Kurt, O. Noury and N. Weiss, Carbon , 2002, 40 , 1715–1728 CrossRef CAS .
- B. J. Landi, M. J. Ganter, C. D. Cress, R. A. DiLeo and R. P. Raffaelle, Energy Environ. Sci. , 2009, 2 , 638 RSC .
- S. Mallakpour and E. Khadem, Chem. Eng. J. , 2016, 302 , 344–367 CrossRef CAS .
- M. W. Chik, Z. Hussain, M. Zulkefeli, M. Tripathy, S. Kumar, A. B. A. Majeed and K. Byrappa, Drug Delivery Transl. Res. , 2019, 9 , 578–594 CrossRef CAS PubMed .
- W. Z. Yuan, Y. Mao, H. Zhao, J. Z. Sun, H. P. Xu, J. K. Jin, Q. Zheng and B. Z. Tang, Macromolecules , 2008, 41 , 701–707 CrossRef CAS .
- C. A. Dyke and J. M. Tour, J. Phys. Chem. A , 2004, 108 , 11151–11159 CrossRef CAS .
- S. Singh, S. Vardharajula, P. Tiwari, E. Eroğlu, K. Vig, V. Dennis and S. Z. Ali, Int. J. Nanomed. , 2012, 7 , 5361 CrossRef PubMed .
- K. Zare, V. K. Gupta, O. Moradi, A. S. H. Makhlouf, M. Sillanpää, M. N. Nadagouda, H. Sadegh, R. Shahryari-ghoshekandi, A. Pal, Z. Wang, I. Tyagi and M. Kazemi, J. Nanostruct. Chem. , 2015, 5 , 227–236 CrossRef CAS .
- E. Vázquez and M. Prato, Pure Appl. Chem. , 2010, 82 , 853–861 Search PubMed .
- A. Setaro, M. Adeli, M. Glaeske, D. Przyrembel, T. Bisswanger, G. Gordeev, F. Maschietto, A. Faghani, B. Paulus, M. Weinelt, R. Arenal, R. Haag and S. Reich, Nat. Commun. , 2017, 8 , 14281 CrossRef CAS PubMed .
- P. Bilalis, D. Katsigiannopoulos, A. Avgeropoulos and G. Sakellariou, RSC Adv. , 2014, 4 , 2911–2934 RSC .
- Y. Zhou, Y. Fang and R. Ramasamy, Sensors , 2019, 19 , 392 CrossRef PubMed .
- T. Morishita, M. Matsushita, Y. Katagiri and K. Fukumori, Carbon , 2009, 47 , 2716–2726 CrossRef CAS .
- M. S. Ata, R. Poon, A. M. Syed, J. Milne and I. Zhitomirsky, Carbon , 2018, 130 , 584–598 CrossRef CAS .
- N. Alzate-Carvajal, L. M. Bolivar-Pineda, V. Meza-Laguna, V. A. Basiuk, E. V. Basiuk and E. A. Baranova, ChemElectroChem , 2020, 7 , 428–436 CrossRef CAS .
- G. Liu, W. Jin and N. Xu, Chem. Soc. Rev. , 2015, 44 , 5016–5030 RSC .
- N. N. Anh, N. Van Chuc, B. H. Thang, P. Van Nhat, N. Hao, D. D. Phuong, P. N. Minh, T. Subramani, N. Fukata and P. Van Trinh, Global Challenges , 2020, 2000010 CrossRef PubMed .
- G. K. Dalapati, S. Masudy-Panah, R. S. Moakhar, S. Chakrabortty, S. Ghosh, A. Kushwaha, R. Katal, C. S. Chua, G. Xiao, S. Tripathy and S. Ramakrishna, Global Challenges , 2020, 4 , 1900087 CrossRef PubMed .
- A. K. Geim and K. S. Novoselov, Nat. Mater. , 2007, 6 , 183–191 CrossRef CAS PubMed .
- A. K. Geim, Science , 2009, 324 , 1530–1534 CrossRef CAS PubMed .
- S. Ghosh, I. Calizo, D. Teweldebrhan, E. P. Pokatilov, D. L. Nika, A. A. Balandin, W. Bao, F. Miao and C. N. Lau, Appl. Phys. Lett. , 2008, 92 , 151911 CrossRef .
- A. A. Balandin, S. Ghosh, W. Bao, I. Calizo, D. Teweldebrhan, F. Miao and C. N. Lau, Nano Lett. , 2008, 8 , 902–907 CrossRef CAS PubMed .
- K. I. Bolotin, K. J. Sikes, Z. Jiang, M. Klima, G. Fudenberg, J. Hone, P. Kim and H. L. Stormer, Solid State Commun. , 2008, 146 , 351–355 CrossRef CAS .
- N. Baig, A.-N. Kawde and M. Ibrahim, Mater. Adv. , 2020, 1 , 783–793, 10.1039/D0MA00322K .
- D. Konios, M. M. Stylianakis, E. Stratakis and E. Kymakis, J. Colloid Interface Sci. , 2014, 430 , 108–112 CrossRef CAS PubMed .
- S. Stankovich, D. A. Dikin, R. D. Piner, K. A. Kohlhaas, A. Kleinhammes, Y. Jia, Y. Wu, S. T. Nguyen and R. S. Ruoff, Carbon , 2007, 45 , 1558–1565 CrossRef CAS .
- J. Chen, Y. Zhang, M. Zhang, B. Yao, Y. Li, L. Huang, C. Li and G. Shi, Chem. Sci. , 2016, 7 , 1874–1881 RSC .
- M. Pumera, Electrochem. Commun. , 2013, 36 , 14–18 CrossRef CAS .
- F. Perreault, A. Fonseca de Faria and M. Elimelech, Chem. Soc. Rev. , 2015, 44 , 5861–5896 RSC .
- A. Pulido, P. Concepción, M. Boronat, C. Botas, P. Alvarez, R. Menendez and A. Corma, J. Mater. Chem. , 2012, 22 , 51–56 RSC .
- N. Baig and T. A. Saleh, Microchim. Acta , 2018, 185 , 283 CrossRef PubMed .
- A. T. Smith, A. M. LaChance, S. Zeng, B. Liu and L. Sun, Nano Mater. Sci. , 2019, 1 , 31–47 CrossRef .
- S. Pei and H.-M. Cheng, Carbon , 2012, 50 , 3210–3228 CrossRef CAS .
- N. Baig and A.-N. Kawde, RSC Adv. , 2016, 6 , 80756–80765 RSC .
- K. K. H. De Silva, H. H. Huang, R. K. Joshi and M. Yoshimura, Carbon , 2017, 119 , 190–199 CrossRef CAS .
- N. Baig, A. Kawde and M. Ibrahim, ChemistrySelect , 2019, 4 , 1640–1649 CrossRef CAS .
- B. Wang, T. Ruan, Y. Chen, F. Jin, L. Peng, Y. Zhou, D. Wang and S. Dou, Energy Storage Mater. , 2020, 24 , 22–51 CrossRef .
- W. Lv, Z. Li, Y. Deng, Q.-H. Yang and F. Kang, Energy Storage Mater. , 2016, 2 , 107–138 CrossRef .
- G. Li, Y. Xia, Y. Tian, Y. Wu, J. Liu, Q. He and D. Chen, J. Electrochem. Soc. , 2019, 166 , B881–B895 CrossRef CAS .
- N. Baig and A.-N. Kawde, Anal. Methods , 2015, 7 , 9535–9541 RSC .
- C. I. L. Justino, A. R. Gomes, A. C. Freitas, A. C. Duarte and T. A. P. Rocha-Santos, TrAC, Trends Anal. Chem. , 2017, 91 , 53–66 CrossRef CAS .
- Q. He, S. Wu, Z. Yin and H. Zhang, Chem. Sci. , 2012, 3 , 1764 RSC .
- H. Yoon, J. Nah, H. Kim, S. Ko, M. Sharifuzzaman, S. C. Barman, X. Xuan, J. Kim and J. Y. Park, Sens. Actuators, B , 2020, 311 , 127866 CrossRef CAS .
- T.-H. Han, Y. Lee, M.-R. Choi, S.-H. Woo, S.-H. Bae, B. H. Hong, J.-H. Ahn and T.-W. Lee, Nat. Photonics , 2012, 6 , 105–110 CrossRef CAS .
- M. Xu, J. Qi, F. Li, X. Liao, S. Liu and Y. Zhang, RSC Adv. , 2017, 7 , 30506–30512 RSC .
- A. Kaidarova, N. Alsharif, B. N. M. Oliveira, M. Marengo, N. R. Geraldi, C. M. Duarte and J. Kosel, Global Challenges , 2020, 4 , 2000001 CrossRef .
- V. H. R. Souza, M. M. Oliveira and A. J. G. Zarbin, J. Power Sources , 2017, 348 , 87–93 CrossRef CAS .
- Y. Zhong, X. Zhang, Y. He, H. Peng, G. Wang and G. Xin, Adv. Funct. Mater. , 2018, 28 , 1801998 CrossRef .
- Mandeep, A. Gulati and R. Kakkar, Chem. Eng. Res. Des. , 2020, 153 , 21–36 CrossRef CAS .
- T. A. Saleh, N. Baig, F. I. Alghunaimi and N. W. Aljuryyed, RSC Adv. , 2020, 10 , 5088–5097 RSC .
- N. Baig, F. I. Alghunaimi, H. S. Dossary and T. A. Saleh, Process Saf. Environ. Prot. , 2019, 123 , 327–334 CrossRef CAS .
- N. Baig, Ihsanullah, M. Sajid and T. A. Saleh, J. Environ. Manage. , 2019, 244 , 370–382 CrossRef CAS PubMed .
- J. Bu, L. Yuan, N. Zhang, D. Liu, Y. Meng and X. Peng, Diamond Relat. Mater. , 2020, 101 , 107604 CrossRef CAS .
- V. V. Danilenko, Phys. Solid State , 2004, 46 , 595–599 CrossRef CAS .
- V. N. Mochalin, O. Shenderova, D. Ho and Y. Gogotsi, Nat. Nanotechnol. , 2012, 7 , 11–23 CrossRef CAS PubMed .
- Y. Zhang, K. Y. Rhee, D. Hui and S.-J. Park, Composites, Part B , 2018, 143 , 19–27 CrossRef CAS .
- T. L. Daulton, M. A. Kirk, R. S. Lewis and L. E. Rehn, Nucl. Instrum. Methods Phys. Res., Sect. B , 2001, 175–177 , 12–20 CrossRef CAS .
- J.-P. Boudou, P. A. Curmi, F. Jelezko, J. Wrachtrup, P. Aubert, M. Sennour, G. Balasubramanian, R. Reuter, A. Thorel and E. Gaffet, Nanotechnology , 2009, 20 , 235602 CrossRef PubMed .
- S. Welz, Y. Gogotsi and M. J. McNallan, J. Appl. Phys. , 2003, 93 , 4207–4214 CrossRef CAS .
- F. Fendrych, A. Taylor, L. Peksa, I. Kratochvilova, J. Vlcek, V. Rezacova, V. Petrak, Z. Kluiber, L. Fekete, M. Liehr and M. Nesladek, J. Phys. D: Appl. Phys. , 2010, 43 , 374018 CrossRef .
- D. Amans, A.-C. Chenus, G. Ledoux, C. Dujardin, C. Reynaud, O. Sublemontier, K. Masenelli-Varlot and O. Guillois, Diamond Relat. Mater. , 2009, 18 , 177–180 CrossRef CAS .
- S. Kumar, M. Nehra, D. Kedia, N. Dilbaghi, K. Tankeshwar and K.-H. Kim, Carbon , 2019, 143 , 678–699 CrossRef CAS .
- D. H. Jariwala, D. Patel and S. Wairkar, Mater. Sci. Eng., C , 2020, 113 , 110996 CrossRef CAS PubMed .
- I. Neitzel, V. N. Mochalin and Y. Gogotsi, Nanodiamonds , Elsevier, 2017, pp. 365–390 Search PubMed .
- H. Wang and Y. Cui, Carbon Energy , 2019, 1 , 13–18 CrossRef .
- H. Lee, H. Kim, S. Weon, W. Choi, Y. S. Hwang, J. Seo, C. Lee and J.-H. Kim, Environ. Sci. Technol. , 2016, 50 , 10134–10142 CrossRef CAS PubMed .
- N. A. Zambianco, T. A. Silva, H. Zanin, O. Fatibello-Filho and B. C. Janegitz, Diamond Relat. Mater. , 2019, 98 , 107512 CrossRef CAS .
- H. Lai, M. H. Stenzel and P. Xiao, Int. Mater. Rev. , 2020, 65 , 189–225 CrossRef CAS .
- U. T. Uthappa, O. R. Arvind, G. Sriram, D. Losic, H.-Y. Jung, M. Kigga and M. D. Kurkuri, J. Drug Delivery Sci. Technol. , 2020, 60 , 101993 CrossRef CAS .
- X. Xu, R. Ray, Y. Gu, H. J. Ploehn, L. Gearheart, K. Raker and W. A. Scrivens, J. Am. Chem. Soc. , 2004, 126 , 12736–12737 CrossRef CAS PubMed .
- J. Shen, Y. Zhu, X. Yang and C. Li, Chem. Commun. , 2012, 48 , 3686 RSC .
- X. Wang, Y. Feng, P. Dong and J. Huang, Front. Chem. , 2019, 7 , 671 CrossRef CAS PubMed .
- P. Karfa, S. De, K. C. Majhi, R. Madhuri and P. K. Sharma, Comprehensive Nanoscience and Nanotechnology , Elsevier, 2019, vol. 1–5, pp. 123–144 Search PubMed .
- M. Li, T. Chen, J. J. Gooding and J. Liu, ACS Sens. , 2019, 4 , 1732–1748 CrossRef CAS PubMed .
- P. Devi, S. Saini and K.-H. Kim, Biosens. Bioelectron. , 2019, 141 , 111158 CrossRef CAS PubMed .
- K. J. Mintz, Y. Zhou and R. M. Leblanc, Nanoscale , 2019, 11 , 4634–4652 RSC .
- H. Liu, T. Ye and C. Mao, Angew. Chem., Int. Ed. , 2007, 46 , 6473–6475 CrossRef CAS PubMed .
- D. Pan, J. Zhang, Z. Li, C. Wu, X. Yan and M. Wu, Chem. Commun. , 2010, 46 , 3681 RSC .
- R. Liu, D. Wu, S. Liu, K. Koynov, W. Knoll and Q. Li, Angew. Chem., Int. Ed. , 2009, 48 , 4598–4601 CrossRef CAS PubMed .
- Y. Dong, N. Zhou, X. Lin, J. Lin, Y. Chi and G. Chen, Chem. Mater. , 2010, 22 , 5895–5899 CrossRef CAS .
- J. Zhou, C. Booker, R. Li, X. Zhou, T.-K. Sham, X. Sun and Z. Ding, J. Am. Chem. Soc. , 2007, 129 , 744–745 CrossRef CAS PubMed .
- J. C. G. Esteves da Silva and H. M. R. Gonçalves, TrAC, Trends Anal. Chem. , 2011, 30 , 1327–1336 CrossRef CAS .
- Y. Wang and A. Hu, J. Mater. Chem. C , 2014, 2 , 6921 RSC .
- S. N. Baker and G. A. Baker, Angew. Chem., Int. Ed. , 2010, 49 , 6726–6744 CrossRef CAS PubMed .
- Y. Li and S. Han, Microchem. J. , 2020, 154 , 104638 CrossRef CAS .
- J. Jiang, Y. He, S. Li and H. Cui, Chem. Commun. , 2012, 48 , 9634 RSC .
- Z.-F. Pu, Q.-L. Wen, Y.-J. Yang, X.-M. Cui, J. Ling, P. Liu and Q.-E. Cao, Spectrochim. Acta, Part A , 2020, 229 , 117944 CrossRef CAS PubMed .
- L. Cao, X. Wang, M. J. Meziani, F. Lu, H. Wang, P. G. Luo, Y. Lin, B. A. Harruff, L. M. Veca, D. Murray, S.-Y. Xie and Y.-P. Sun, J. Am. Chem. Soc. , 2007, 129 , 11318–11319 CrossRef CAS PubMed .
- K. Cheng, R. Qi, S. Lan, H. Wang, X. Zheng, C. Liu, D. Jia, L. Cao and D. Wang, Dyes Pigm. , 2020, 174 , 108106 CrossRef CAS .
- F. Copur, N. Bekar, E. Zor, S. Alpaydin and H. Bingol, Sens. Actuators, B , 2019, 279 , 305–312 CrossRef CAS .
- Z. Nan, C. Hao, X. Zhang, H. Liu and R. Sun, Spectrochim. Acta, Part A , 2020, 228 , 117717 CrossRef CAS PubMed .
- C. Wang, H. Shi, M. Yang, Y. Yan, E. Liu, Z. Ji and J. Fan, J. Photochem. Photobiol., A , 2020, 391 , 112374 CrossRef CAS .
- W. Li, S. Huang, H. Wen, Y. Luo, J. Cheng, Z. Jia, P. Han and W. Xue, Anal. Bioanal. Chem. , 2020, 412 , 993–1002 CrossRef CAS PubMed .
- X. Tian, H. Peng, Y. Li, C. Yang, Z. Zhou and Y. Wang, Sens. Actuators, B , 2017, 243 , 1002–1009 CrossRef CAS .
- G. Han, J. Zhao, R. Zhang, X. Tian, Z. Liu, A. Wang, R. Liu, B. Liu, M. Han, X. Gao and Z. Zhang, Angew. Chem., Int. Ed. , 2019, 58 , 7087–7091 CrossRef CAS PubMed .
- H. Li, F.-Q. Shao, H. Huang, J.-J. Feng and A.-J. Wang, Sens. Actuators, B , 2016, 226 , 506–511 CrossRef CAS .
- Y. Sun, S. Zheng, L. Liu, Y. Kong, A. Zhang, K. Xu and C. Han, Nanoscale Res. Lett. , 2020, 15 , 55 CrossRef CAS PubMed .
- Y. Wei, L. Chen, J. Wang, X. Liu, Y. Yang and S. Yu, Opt. Mater. , 2020, 100 , 109647 CrossRef CAS .
- T. Song, Y. Zhao, T. Wang, J. Li, Z. Jiang and P. Yang, J. Fluoresc. , 2020, 30 , 81–89 CrossRef CAS PubMed .
- G. Huang, Y. Lin, L. Zhang, Z. Yan, Y. Wang and Y. Liu, Sci. Rep. , 2019, 9 , 19651 CrossRef CAS PubMed .
- X. Wang, L. Cao, F. Lu, M. J. Meziani, H. Li, G. Qi, B. Zhou, B. A. Harruff, F. Kermarrec and Y.-P. Sun, Chem. Commun. , 2009, 3774 RSC .
- Y. Li, B.-P. Zhang, J.-X. Zhao, Z.-H. Ge, X.-K. Zhao and L. Zou, Appl. Surf. Sci. , 2013, 279 , 367–373 CrossRef CAS .
- Y. Zhang, Y. Zhao, Z. Xu, H. Su, X. Bian, S. Zhang, X. Dong, L. Zeng, T. Zeng, M. Feng, L. Li and V. K. Sharma, Appl. Catal., B , 2020, 262 , 118306 CrossRef CAS .
- L. Zhang, J. Zhang, Y. Xia, M. Xun, H. Chen, X. Liu and X. Yin, Int. J. Mol. Sci. , 2020, 21 , 1052 CrossRef CAS PubMed .
- Y.-Y. Li, Y. Si, B.-X. Zhou, T. Huang, W.-Q. Huang, W. Hu, A. Pan, X. Fan and G.-F. Huang, Nanoscale , 2020, 12 , 3135–3145 RSC .
- N. Karousis, I. Suarez-Martinez, C. P. Ewels and N. Tagmatarchis, Chem. Rev. , 2016, 116 , 4850–4883 CrossRef CAS PubMed .
- M. Zhang and M. Yudasaka, Carbon Nanostructures , Springer International Publishing, 2016, pp. 77–107 Search PubMed .
- S. Iijima, M. Yudasaka, R. Yamada, S. Bandow, K. Suenaga, F. Kokai and K. Takahashi, Chem. Phys. Lett. , 1999, 309 , 165–170 CrossRef CAS .
- S. Zhu and G. Xu, Nanoscale , 2010, 2 , 2538 RSC .
- J. Fan, M. Yudasaka, J. Miyawaki, K. Ajima, K. Murata and S. Iijima, J. Phys. Chem. B , 2006, 110 , 1587–1591 CrossRef CAS PubMed .
- Z. Zhang, S. Han, C. Wang, J. Li and G. Xu, Nanomaterials , 2015, 5 , 1732–1755 CrossRef CAS PubMed .
- Z. Liu, W. Zhang, W. Qi, W. Gao, S. Hanif, M. Saqib and G. Xu, Chem. Commun. , 2015, 51 , 4256–4258 RSC .
- T. Ohba, K. Murata, K. Kaneko, W. A. Steele, F. Kokai, K. Takahashi, D. Kasuya, M. Yudasaka and S. Iijima, Nano Lett. , 2001, 1 , 371–373 CrossRef CAS .
- D. Ebenezer, M. Jagannatham, M. S. Lahari, P. Bhuwaneshswar and H. Prathap, Diamond Relat. Mater. , 2016, 70 , 26–32 CrossRef CAS .
- H. Wang, L. Pan, Y. Liu, Y. Ye and S. Yao, J. Electroanal. Chem. , 2020, 862 , 113955 CrossRef CAS .
- T. Yoshitake, Y. Shimakawa, S. Kuroshima, H. Kimura, T. Ichihashi, Y. Kubo, D. Kasuya, K. Takahashi, F. Kokai, M. Yudasaka and S. Iijima, Phys. B , 2002, 323 , 124–126 CrossRef CAS .
- A. Thomas, Nat. Commun. , 2020, 11 , 4985 CrossRef CAS .
- R. W. Derlet and T. E. Albertson, West. J. Med. , 1986, 145 , 493–496 CAS .
- R. Mishra, J. Militky and M. Venkataraman, Nanotechnology in Textiles: Theory and Application , 2018, pp. 311–353 Search PubMed .
- N. Pal, Adv. Colloid Interface Sci. , 2020, 280 , 102156 CrossRef CAS PubMed .
- M. E. Davis, Nature , 2002, 417 , 813–821 CrossRef CAS .
- S. Polarz and B. Smarsly, J. Nanosci. Nanotechnol. , 2002, 2 , 581–612 CrossRef CAS .
- B. Szczęśniak, S. Borysiuk, J. Choma and M. Jaroniec, Mater. Horiz. , 2020, 7 , 1457–1473 RSC .
- D. Chen, L. Wei, J. Li and Q. Wu, J. Energy Storage , 2020, 30 , 101525 CrossRef .
- L. Zhang and M. Jaroniec, Chem. Soc. Rev. , 2020, 49 , 6039–6055 RSC .
- Y. Li, H. Cao and J. Yu, ACS Nano , 2018, 12 , 4096–4104 CrossRef CAS .
- Z. Yang, Z. Xie, H. Liu, F. Yan and H. Ju, Adv. Funct. Mater. , 2008, 18 , 3991–3998 CrossRef CAS .
- S. Bagheri, M. M. Amini, M. Behbahani and G. Rabiee, Microchem. J. , 2019, 145 , 460–469 CrossRef CAS .
- Y. Zhou, G. Quan, Q. Wu, X. Zhang, B. Niu, B. Wu, Y. Huang, X. Pan and C. Wu, Acta Pharm. Sin. B , 2018, 8 , 165–177 CrossRef PubMed .
- X. Wang, C. Li, N. Fan, J. Li, Z. He and J. Sun, Mater. Sci. Eng., C , 2017, 78 , 370–375 CrossRef CAS PubMed .
- V. Zeleňák, D. Halamová, M. Almáši, L. Žid, A. Zeleňáková and O. Kapusta, Appl. Surf. Sci. , 2018, 443 , 525–534 CrossRef .
- Y. Wang, Q. Zhao, N. Han, L. Bai, J. Li, J. Liu, E. Che, L. Hu, Q. Zhang, T. Jiang and S. Wang, Nanomedicine , 2015, 11 , 313–327 CrossRef CAS PubMed .
- K. Na and G. A. Somorjai, Catal. Lett. , 2015, 145 , 193–213 CrossRef CAS .
- K. Na, M. Choi and R. Ryoo, Microporous Mesoporous Mater. , 2013, 166 , 3–19 CrossRef CAS .
- S. Zhang, Z. Zhang, H. Li, Z. Yu, Q. Huang, Z. Qiao, L. Zong, L. Yan, J. Li and J. Kang, Chem. Eng. J. , 2020, 383 , 123097 CrossRef CAS .
- A. Mohammadi Zardkhoshoui and S. S. Hosseiny Davarani, Dalton Trans. , 2020, 49 , 10028–10041 RSC .
- S. Ouyang, P. Li, H. Xu, H. Tong, L. Liu and J. Ye, ACS Appl. Mater. Interfaces , 2014, 6 , 22726–22732 CrossRef CAS PubMed .
- T. Xu, H. Zheng and P. Zhang, J. Hazard. Mater. , 2020, 388 , 121746 CrossRef CAS PubMed .
- W. Luc and F. Jiao, Acc. Chem. Res. , 2016, 49 , 1351–1358 CrossRef CAS PubMed .
- T. Zhang, M. Paulose, R. Neupane, L. A. Schaffer, D. B. Rana, J. Su, L. Guo and O. K. Varghese, Sol. Energy Mater. Sol. Cells , 2020, 209 , 110472 CrossRef CAS .
- G. Schmid, J. Mater. Chem. , 2002, 12 , 1231–1238 RSC .
- A. Larsson, G. Abbondanza, W. Linpé, F. Carlà, P. Mousley, C. Hetherington, E. Lundgren and G. S. Harlow, J. Electrochem. Soc. , 2020, 167 , 122514 CrossRef CAS .
- P. G. Schiavi, P. Altimari, A. Rubino and F. Pagnanelli, Electrochim. Acta , 2018, 259 , 711–722 CrossRef CAS .
- A. Yadav, M. S. Bobji and S. J. Bull, J. Nanosci. Nanotechnol. , 2019, 19 , 4254–4259 CrossRef CAS PubMed .
- F. Yang, J. Tang, B. Shao, S. Gao and X. Liu, Nano-Struct. Nano-Objects , 2019, 18 , 100287 CrossRef .
- G. Rajeev, B. Prieto Simon, L. F. Marsal and N. H. Voelcker, Adv. Healthcare Mater. , 2018, 7 , 1700904 CrossRef PubMed .
- M. R. Benzigar, S. N. Talapaneni, S. Joseph, K. Ramadass, G. Singh, J. Scaranto, U. Ravon, K. Al-Bahily and A. Vinu, Chem. Soc. Rev. , 2018, 47 , 2680–2721 RSC .
- N. Baig and T. A. Saleh, Composites, Part B , 2019, 173 , 106805 CrossRef .
- N. Baig and T. A. Saleh, Global Challenges , 2019, 3 , 1800115 CrossRef PubMed .
- N. Baig and T. A. Saleh, Chem. – Asian J. , 2021, 16 , 329–341, DOI: 10.1002/asia.202001368 .
- S. Kundu, I. H. Chowdhury and M. K. Naskar, ACS Omega , 2018, 3 , 9888–9898 CrossRef CAS PubMed .
- T. A. Saleh, N. Baig, H. A. Othman and A. M. Al Harith, Chem. Eng. J. , 2021, 407 , 126216 CrossRef CAS .
- B. Szczęśniak, J. Phuriragpitikhon, J. Choma and M. Jaroniec, J. Mater. Chem. A , 2020, 8 , 18464–18491 RSC .
- Z. Bi, Q. Kong, Y. Cao, G. Sun, F. Su, X. Wei, X. Li, A. Ahmad, L. Xie and C.-M. Chen, J. Mater. Chem. A , 2019, 7 , 16028–16045 RSC .
- Z. Li, D. Guo, Y. Liu, H. Wang and L. Wang, Chem. Eng. J. , 2020, 397 , 125418 CrossRef CAS .
- Z. Li, J. Xu, D. Sun, T. Lin and F. Huang, ACS Appl. Nano Mater. , 2020, 3 , 1564–1570 CrossRef CAS .
- A. O. Abo El Naga, S. A. Shaban and F. Y. A. El Kady, J. Taiwan Inst. Chem. Eng. , 2018, 93 , 363–373 CrossRef CAS .
- C. Jiao, Y. Wang, M. Li, Q. Wu, C. Wang and Z. Wang, J. Magn. Magn. Mater. , 2016, 407 , 24–30 CrossRef CAS .
- M. Anbia and S. Amirmahmoodi, Arabian J. Chem. , 2016, 9 , S319–S325 CrossRef CAS .
- H. Wang, J. Jia, P. Song, Q. Wang, D. Li, S. Min, C. Qian, L. Wang, Y. F. Li, C. Ma, T. Wu, J. Yuan, M. Antonietti and G. A. Ozin, Angew. Chem. , 2017, 129 , 7955–7960 CrossRef .
- Y. Zhang, H. Sun, Y. Qiu, E. Zhang, T. Ma, G. Gao, C. Cao, Z. Ma and P. Hu, Energy , 2018, 165 , 537–548 CrossRef CAS .
- H. Luo, W.-J. Jiang, Y. Zhang, S. Niu, T. Tang, L.-B. Huang, Y.-Y. Chen, Z. Wei and J.-S. Hu, Carbon , 2018, 128 , 97–105 CrossRef CAS .
- J. Tang, J. Liu, N. L. Torad, T. Kimura and Y. Yamauchi, Nano Today , 2014, 9 , 305–323 CrossRef CAS .
- R. Brilmayer, C. Förster, L. Zhao and A. Andrieu-Brunsen, Curr. Opin. Biotechnol. , 2020, 63 , 200–209 CrossRef CAS .
- O. K. Farha, A. Özgür Yazaydın, I. Eryazici, C. D. Malliakas, B. G. Hauser, M. G. Kanatzidis, S. T. Nguyen, R. Q. Snurr and J. T. Hupp, Nat. Chem. , 2010, 2 , 944–948 CrossRef CAS PubMed .
- O. K. Farha, I. Eryazici, N. C. Jeong, B. G. Hauser, C. E. Wilmer, A. A. Sarjeant, R. Q. Snurr, S. T. Nguyen, A. Ö. Yazaydın and J. T. Hupp, J. Am. Chem. Soc. , 2012, 134 , 15016–15021 CrossRef CAS PubMed .
- I. M. Hönicke, I. Senkovska, V. Bon, I. A. Baburin, N. Bönisch, S. Raschke, J. D. Evans and S. Kaskel, Angew. Chem., Int. Ed. , 2018, 57 , 13780–13783 CrossRef PubMed .
- V. Chernikova, O. Shekhah, Y. Belmabkhout and M. Eddaoudi, ACS Appl. Nano Mater. , 2020, 3 , 6432–6439 CrossRef CAS .
- H. A. Patel, S. Hyun Je, J. Park, D. P. Chen, Y. Jung, C. T. Yavuz and A. Coskun, Nat. Commun. , 2013, 4 , 1357 CrossRef PubMed .
- Y. Zhang, S. Wei, F. Liu, Y. Du, S. Liu, Y. Ji, T. Yokoi, T. Tatsumi and F.-S. Xiao, Nano Today , 2009, 4 , 135–142 CrossRef CAS .
- M. Rose, ChemCatChem , 2014, 6 , 1166–1182 CAS .
- N. Wang, Q. Sun and J. Yu, Adv. Mater. , 2019, 31 , 1803966 CrossRef PubMed .
- C. R. Martin, Science , 1994, 266 , 1961–1966 CrossRef CAS PubMed .
- H. Zhao, X. Chen, G. Wang, Y. Qiu and L. Guo, 2D Mater. , 2019, 6 , 032002, DOI: 10.1088/2053-1583/ab1169 .
- C. Tan, X. Cao, X.-J. Wu, Q. He, J. Yang, X. Zhang, J. Chen, W. Zhao, S. Han, G.-H. Nam, M. Sindoro and H. Zhang, Chem. Rev. , 2017, 117 , 6225–6331 CrossRef CAS PubMed .
- A. A. F. K. S. Novoselov, A. K. Geim, S. V. Morozov, D. Jiang, Y. Zhang, S. V. Dubonos and I. V. Grigorieva, Science , 2004, 306 , 666–669 CrossRef PubMed .
- F. Bai, L. Xu, X. Zhai, X. Chen and W. Yang, Adv. Energy Mater. , 2020, 10 , 1–19 Search PubMed .
- K. Takeda and K. Shiraishi, Teorìâ ta Metod. Fìzičnogo Vihovannâ , 1994, 50 , 14916–14922 CAS .
- G. G. Guzmán-Verri and L. C. Lew Yan Voon, Phys. Rev. B: Condens. Matter Mater. Phys. , 2007, 76 , 075131, DOI: 10.1103/PhysRevB.76.075131 .
- S. Cahangirov, M. Topsakal, E. Aktürk, H. Šahin and S. Ciraci, Phys. Rev. Lett. , 2009, 102 , 1–4 CrossRef PubMed .
- N. D. Drummond, V. Zólyomi and V. I. Fal’Ko, Phys. Rev. B: Condens. Matter Mater. Phys. , 2012, 85 , 1–7 CrossRef .
- Z. Ni, Q. Liu, K. Tang, J. Zheng, J. Zhou, R. Qin, Z. Gao, D. Yu and J. Lu, Nano Lett. , 2012, 12 , 113–118 CrossRef CAS PubMed .
- C. C. Liu, W. Feng and Y. Yao, Phys. Rev. Lett. , 2011, 107 , 1–4 Search PubMed .
- F. Liu, C. C. Liu, K. Wu, F. Yang and Y. Yao, Phys. Rev. Lett. , 2013, 111 , 1–5 Search PubMed .
- C. Xu, G. Luo, Q. Liu, J. Zheng, Z. Zhang, S. Nagase, Z. Gao and J. Lu, Nanoscale , 2012, 4 , 3111–3117 RSC .
- J. Zhao, H. Liu, Z. Yu, R. Quhe, S. Zhou, Y. Wang, C. C. Liu, H. Zhong, N. Han, J. Lu, Y. Yao and K. Wu, Prog. Mater. Sci. , 2016, 83 , 24–151 CrossRef CAS .
- M. De Crescenzi, P. Castrucci, M. Scarselli, M. Diociaiuti, P. S. Chaudhari, C. Balasubramanian, T. M. Bhave and S. V. Bhoraskar, Appl. Phys. Lett. , 2005, 86 , 1–3 CrossRef .
- S. Yamada and H. Fujiki, Jpn. J. Appl. Phys., Part 2 , 2006, 45 , 18–21 CrossRef .
- H. Nakano, T. Mitsuoka, M. Harada, K. Horibuchi, H. Nozaki, N. Takahashi, T. Nonaka, Y. Seno and H. Nakamura, Angew. Chem., Int. Ed. , 2006, 45 , 6303–6306 CrossRef CAS PubMed .
- P. De Padova, C. Quaresima, C. Ottaviani, P. M. Sheverdyaeva, P. Moras, C. Carbone, D. Topwal, B. Olivieri, A. Kara, H. Oughaddou, B. Aufray and G. Le Lay, Appl. Phys. Lett. , 2010, 96 , 1–4 CrossRef .
- B. Aufray, A. Kara, Ś. Vizzini, H. Oughaddou, C. Ĺandri, B. Ealet and G. Le-Lay, Appl. Phys. Lett. , 2010, 96 , 1–4 CrossRef .
- C. Léandri, H. Oughaddou, B. Aufray, J. M. Gay, G. Le Lay, A. Ranguis and Y. Garreau, Surf. Sci. , 2007, 601 , 262–267 CrossRef .
- P. Vogt, P. De Padova, C. Quaresima, J. Avila, E. Frantzeskakis, M. C. Asensio, A. Resta, B. Ealet and G. Le Lay, Phys. Rev. Lett. , 2012, 108 , 155501 CrossRef .
- A. Fleurence, R. Friedlein, T. Ozaki, H. Kawai, Y. Wang and Y. Yamada-Takamura, Phys. Rev. Lett. , 2012, 108 , 1–5 CrossRef PubMed .
- L. Meng, Y. Wang, L. Zhang, S. Du, R. Wu, L. Li, Y. Zhang, G. Li, H. Zhou, W. A. Hofer and H. J. Gao, Nano Lett. , 2013, 13 , 685–690 CrossRef CAS PubMed .
- T. Aizawa, S. Suehara and S. Otani, J. Phys. Chem. C , 2014, 118 , 23049–23057 CrossRef CAS .
- D. Chiappe, E. Scalise, E. Cinquanta, C. Grazianetti, B. Van Den Broek, M. Fanciulli, M. Houssa and A. Molle, Adv. Mater. , 2014, 26 , 2096–2101 CrossRef CAS PubMed .
- B. Feng, Z. Ding, S. Meng, Y. Yao, X. He, P. Cheng, L. Chen and K. Wu, Nano Lett. , 2012, 12 , 3507–3511 CrossRef CAS PubMed .
- D. Chiappe, C. Grazianetti, G. Tallarida, M. Fanciulli and A. Molle, Adv. Mater. , 2012, 24 , 5088–5093 CrossRef CAS PubMed .
- P. De Padova, O. Kubo, B. Olivieri, C. Quaresima, T. Nakayama, M. Aono and G. Le Lay, Nano Lett. , 2012, 12 , 5500–5503 CrossRef CAS PubMed .
- L. Tao, E. Cinquanta, D. Chiappe, C. Grazianetti, M. Fanciulli, M. Dubey, A. Molle and D. Akinwande, Nat. Nanotechnol. , 2015, 10 , 227–231 CrossRef CAS PubMed .
- J. Liu, Y. Yang, P. Lyu, P. Nachtigall and Y. Xu, Adv. Mater. , 2018, 30 , 1–7 CAS .
- M. Naguib, V. N. Mochalin, M. W. Barsoum and Y. Gogotsi, Adv. Mater. , 2014, 26 , 992–1005 CrossRef CAS .
- M. Ghidiu, M. R. Lukatskaya, M. Q. Zhao, Y. Gogotsi and M. W. Barsoum, Nature , 2014, 516 , 78–81 CrossRef CAS PubMed .
- X. Sang, Y. Xie, M. W. Lin, M. Alhabeb, K. L. Van Aken, Y. Gogotsi, P. R. C. Kent, K. Xiao and R. R. Unocic, ACS Nano , 2016, 10 , 9193–9200 CrossRef CAS PubMed .
- M. Naguib, M. Kurtoglu, V. Presser, J. Lu, J. Niu, M. Heon, L. Hultman, Y. Gogotsi and M. W. Barsoum, Adv. Mater. , 2011, 23 , 4248–4253 CrossRef CAS PubMed .
- J. Pang, R. G. Mendes, A. Bachmatiuk, L. Zhao, H. Q. Ta, T. Gemming, H. Liu, Z. Liu and M. H. Rummeli, Chem. Soc. Rev. , 2019, 48 , 72–133 RSC .
- Z. Li and Y. Wu, Small , 2019, 15 , 1–10 Search PubMed .
- J. Ran, G. Gao, F. T. Li, T. Y. Ma, A. Du and S. Z. Qiao, Nat. Commun. , 2017, 8 , 13907, DOI: 10.1038/ncomms13907 .
- Z. W. Seh, K. D. Fredrickson, B. Anasori, J. Kibsgaard, A. L. Strickler, M. R. Lukatskaya, Y. Gogotsi, T. F. Jaramillo and A. Vojvodic, ACS Energy Lett. , 2016, 1 , 589–594 CrossRef CAS .
- A. Kumar and A. Bhan, Chem. Eng. Sci. , 2019, 197 , 371–378 CrossRef CAS .
- D. Wang, Z. Wang, L. Wang, L. Hu and J. Jin, Nanoscale , 2015, 7 , 17649–17652 RSC .
- J. Abraham, K. S. Vasu, C. D. Williams, K. Gopinadhan, Y. Su, C. T. Cherian, J. Dix, E. Prestat, S. J. Haigh, I. V. Grigorieva, P. Carbone, A. K. Geim and R. R. Nair, Nat. Nanotechnol. , 2017, 12 , 546–550 CrossRef CAS PubMed .
- G. Reina, J. M. González-Domínguez, A. Criado, E. Vázquez, A. Bianco and M. Prato, Chem. Soc. Rev. , 2017, 46 , 4400–4416 RSC .
- Y. Zhang, H. Jiang, Y. Lin, H. Liu, Q. He, C. Wu, T. Duan and L. Song, Adv. Mater. Interfaces , 2018, 5 , 1–9 Search PubMed .
- T. Y. Ma, J. L. Cao, M. Jaroniec and S. Z. Qiao, Angew. Chem. , 2016, 128 , 1150–1154 CrossRef .
- M. Yu, S. Zhou, Z. Wang, J. Zhao and J. Qiu, Nano Energy , 2018, 44 , 181–190 CrossRef CAS .
- N. Hao, Y. Wei, J. Wang, Z. Wang, Z. Zhu, S. Zhao, M. Han and X. Huang, RSC Adv. , 2018, 8 , 20576–20584 RSC .
- L. Zhao, B. Dong, S. Li, L. Zhou, L. Lai, Z. Wang, S. Zhao, M. Han, K. Gao, M. Lu, X. Xie, B. Chen, Z. Liu, X. Wang, H. Zhang, H. Li, J. Liu, H. Zhang, X. Huang and W. Huang, ACS Nano , 2017, 11 , 5800–5807 CrossRef CAS PubMed .
- B. Lyu, Y. Choi, H. Jing, C. Qian, H. Kang, S. Lee and J. H. Cho, Adv. Mater. , 2020, 32 , 2–9 Search PubMed .
- L. Li, Y. Yu, G. J. Ye, Q. Ge, X. Ou, H. Wu, D. Feng, X. H. Chen and Y. Zhang, Nat. Nanotechnol. , 2014, 9 , 372–377 CrossRef CAS PubMed .
- M. Buscema, D. J. Groenendijk, S. I. Blanter, G. A. Steele, H. S. J. Van Der Zant and A. Castellanos-Gomez, Nano Lett. , 2014, 14 , 3347–3352 CrossRef CAS .
- G. Slotman, A. Rudenko, E. Van Veen, M. I. Katsnelson, R. Roldán and S. Yuan, Phys. Rev. B , 2018, 98 , 1–7 CrossRef .
- C. Hao, B. Yang, F. Wen, J. Xiang, L. Li, W. Wang, Z. Zeng, B. Xu, Z. Zhao, Z. Liu and Y. Tian, Adv. Mater. , 2016, 28 , 3194–3201 CrossRef CAS PubMed .
- C. M. Park and H. J. Sohn, Adv. Mater. , 2007, 19 , 2465–2468 CrossRef CAS .
- M. Pumera and Z. Sofer, Adv. Mater. , 2017, 29 , 1605299, DOI: 10.1002/adma.201605299 .
- S. Zhang, M. Xie, F. Li, Z. Yan, Y. Li, E. Kan, W. Liu, Z. Chen and H. Zeng, Angew. Chem., Int. Ed. , 2016, 55 , 1666–1669 CrossRef CAS PubMed .
- M. Wu, H. Fu, L. Zhou, K. Yao and X. C. Zeng, Nano Lett. , 2015, 15 , 3557–3562 CrossRef CAS PubMed .
- P. Ares, F. Aguilar-Galindo, D. Rodríguez-San-Miguel, D. A. Aldave, S. Díaz-Tendero, M. Alcamí, F. Martín, J. Gómez-Herrero and F. Zamora, Adv. Mater. , 2016, 28 , 6332–6336 CrossRef CAS PubMed .
- Y. Wang and Y. Ding, Nanoscale Res. Lett. , 2015, 10 , 1–10 CrossRef PubMed .
- J. Ji, X. Song, J. Liu, Z. Yan, C. Huo, S. Zhang, M. Su, L. Liao, W. Wang, Z. Ni, Y. Hao and H. Zeng, Nat. Commun. , 2016, 7 , 1–9 Search PubMed .
- H. Liu, A. T. Neal, Z. Zhu, Z. Luo, X. Xu, D. Tománek and P. D. Ye, ACS Nano , 2014, 8 , 4033–4041 CrossRef CAS PubMed .
- A. Favron, E. Gaufrès, F. Fossard, A. L. Phaneuf-Laheureux, N. Y. W. Tang, P. L. Lévesque, A. Loiseau, R. Leonelli, S. Francoeur and R. Martel, Nat. Mater. , 2015, 14 , 826–832 CrossRef CAS PubMed .
- G. Abellán, S. Wild, V. Lloret, N. Scheuschner, R. Gillen, U. Mundloch, J. Maultzsch, M. Varela, F. Hauke and A. Hirsch, J. Am. Chem. Soc. , 2017, 139 , 10432–10440 CrossRef PubMed .
- C. Gibaja, D. Rodriguez-San-Miguel, P. Ares, J. Gómez-Herrero, M. Varela, R. Gillen, J. Maultzsch, F. Hauke, A. Hirsch, G. Abellán and F. Zamora, Angew. Chem. , 2016, 128 , 14557–14561 CrossRef .
- O. Ü. Aktürk, V. O. Özçelik and S. Ciraci, Phys. Rev. B: Condens. Matter Mater. Phys. , 2015, 91 , 1–10 CrossRef .
- S. K. Gupta, Y. Sonvane, G. Wang and R. Pandey, Chem. Phys. Lett. , 2015, 641 , 169–172 CrossRef CAS .
- G. Wang, R. Pandey and S. P. Karna, ACS Appl. Mater. Interfaces , 2015, 7 , 11490–11496 CrossRef CAS PubMed .
- S. Zhang, Z. Yan, Y. Li, Z. Chen and H. Zeng, Angew. Chem. , 2015, 127 , 3155–3158 CrossRef .
- C. Kamal and M. Ezawa, Phys. Rev. B: Condens. Matter Mater. Phys. , 2015, 91 , 1–10 CrossRef .
- Z. Zhang, J. Xie, D. Yang, Y. Wang, M. Si and D. Xue, Appl. Phys. Express , 2015, 8 , 055201, DOI: 10.7567/APEX.8.055201 .
- M. S. Khan, A. Srivastava and R. Pandey, RSC Adv. , 2016, 6 , 72634–72642 RSC .
- M. S. Khan, V. Ranjan and A. Srivastava, Proc. – 2015 IEEE Int. Symp. Nanoelectron. Inf. Syst. iNIS 2015 , 2016, 248–251.
- S. Guo, Y. Zhang, Y. Ge, S. Zhang, H. Zeng and H. Zhang, Adv. Mater. , 2019, 31 , 1–19 Search PubMed .
- Y. Liu, T. Wang, J. Robertson, J. Luo, Y. Guo and D. Liu, J. Phys. Chem. C , 2020, 124 , 7441–7448 CrossRef CAS .
- G. Abellán, P. Ares, S. Wild, E. Nuin, C. Neiss, D. R. S. Miguel, P. Segovia, C. Gibaja, E. G. Michel, A. Görling, F. Hauke, J. Gómez-Herrero, A. Hirsch and F. Zamora, Angew. Chem., Int. Ed. , 2017, 56 , 14389–14394 CrossRef PubMed .
- S. Wolff, R. Gillen, M. Assebban, G. Abellán and J. Maultzsch, Phys. Rev. Lett. , 2020, 124 , 126101 CrossRef CAS PubMed .
- T. García-Mendiola, C. Gutiérrez-Sánchez, C. Gibaja, I. Torres, C. Busó-Rogero, F. Pariente, J. Solera, Z. Razavifar, J. J. Palacios, F. Zamora and E. Lorenzo, ACS Appl. Nano Mater. , 2020, 3 , 3625–3633 CrossRef .
- T. Xue, W. Liang, Y. Li, Y. Sun, Y. Xiang, Y. Zhang, Z. Dai, Y. Duo, L. Wu, K. Qi, B. N. Shivananju, L. Zhang, X. Cui, H. Zhang and Q. Bao, Nat. Commun. , 2019, 10 , 1–9 CrossRef PubMed .
- Z. Wang, Z. Wang, Z. Wang, M. Zhao, Y. Zhou, B. Zhao, Y. Miao, P. Liu, Y. Hao, H. Wang, B. Xu, Y. Wu and S. Yin, 2D Mater. , 2019, 6 , 045017, DOI: 10.1088/2053-1583/ab277d .
- E. Martínez-Periñán, M. P. Down, C. Gibaja, E. Lorenzo, F. Zamora and C. E. Banks, Adv. Energy Mater. , 2018, 8 , 1–8 Search PubMed .
- Y. H. Luo, C. Chen, C. He, Y. Y. Zhu, D. L. Hong, X. T. He, P. J. An, H. S. Wu and B. W. Sun, ACS Appl. Mater. Interfaces , 2018, 10 , 28860–28867 CrossRef CAS PubMed .
- A. Abhervé, S. Mañas-Valero, M. Clemente-León and E. Coronado, Chem. Sci. , 2015, 6 , 4665–4673 RSC .
- H. S. Wang, J. Li, J. Y. Li, K. Wang, Y. Ding and X. H. Xia, NPG Asia Mater. , 2017, 9 , 1–9 Search PubMed .
- W. J. Song, Talanta , 2017, 170 , 74–80 CrossRef CAS PubMed .
- Y. Ding, Y. P. Chen, X. Zhang, L. Chen, Z. Dong, H. L. Jiang, H. Xu and H. C. Zhou, J. Am. Chem. Soc. , 2017, 139 , 9136–9139 CrossRef CAS PubMed .
- T. Kambe, R. Sakamoto, K. Hoshiko, K. Takada, M. Miyachi, J. H. Ryu, S. Sasaki, J. Kim, K. Nakazato, M. Takata and H. Nishihara, J. Am. Chem. Soc. , 2013, 135 , 2462–2465 CrossRef CAS PubMed .
- L. Cao, Z. Lin, F. Peng, W. Wang, R. Huang, C. Wang, J. Yan, J. Liang, Z. Zhang, T. Zhang, L. Long, J. Sun and W. Lin, Angew. Chem. , 2016, 128 , 5046–5050 CrossRef .
- T. Rodenas, I. Luz, G. Prieto, B. Seoane, H. Miro, A. Corma, F. Kapteijn, F. X. Llabrés I Xamena and J. Gascon, Nat. Mater. , 2015, 14 , 48–55 CrossRef CAS PubMed .
- S. C. Junggeburth, L. Diehl, S. Werner, V. Duppel, W. Sigle and B. V. Lotsch, J. Am. Chem. Soc. , 2013, 135 , 6157–6164 CrossRef CAS PubMed .
- Y. Zheng, S. Zheng, Y. Xu, H. Xue, C. Liu and H. Pang, Chem. Eng. J. , 2019, 373 , 1319–1328 CrossRef CAS .
- Y. Wang, Y. Liu, H. Wang, W. Liu, Y. Li, J. Zhang, H. Hou and J. Yang, ACS Appl. Energy Mater. , 2019, 2 , 2063–2071 CrossRef CAS .
- C. Li, X. Hu, W. Tong, W. Yan, X. Lou, M. Shen and B. Hu, ACS Appl. Mater. Interfaces , 2017, 9 , 29829–29838 CrossRef CAS PubMed .
- A. J. Clough, J. W. Yoo, M. H. Mecklenburg and S. C. Marinescu, J. Am. Chem. Soc. , 2015, 137 , 118–121 CrossRef CAS PubMed .
- R. Dong, M. Pfeffermann, H. Liang, Z. Zheng, X. Zhu, J. Zhang and X. Feng, Angew. Chem., Int. Ed. , 2015, 54 , 12058–12063 CrossRef CAS PubMed .
- B. C. Patra, S. Khilari, R. N. Manna, S. Mondal, D. Pradhan, A. Pradhan and A. Bhaumik, ACS Catal. , 2017, 7 , 6120–6127 CrossRef CAS .
- Y. Xu, B. Li, S. Zheng, P. Wu, J. Zhan, H. Xue, Q. Xu and H. Pang, J. Mater. Chem. A , 2018, 6 , 22070–22076 RSC .
- G. Hai, X. Jia, K. Zhang, X. Liu, Z. Wu and G. Wang, Nano Energy , 2018, 44 , 345–352 CrossRef CAS .
- M. Jian, H. Liu, T. Williams, J. Ma, H. Wang and X. Zhang, Chem. Commun. , 2017, 53 , 13161–13164 RSC .
- E. M. Miner, T. Fukushima, D. Sheberla, L. Sun, Y. Surendranath and M. Dinca, Nat. Commun. , 2016, 7 , 1–7 Search PubMed .
- K. Zhao, S. Liu, G. Ye, X. Wei, Y. Su, W. Zhu, Z. Zhou and Z. He, ChemSusChem , 2020, 13 , 1556–1567 CrossRef CAS PubMed .
- N. Kornienko, Y. Zhao, C. S. Kley, C. Zhu, D. Kim, S. Lin, C. J. Chang, O. M. Yaghi and P. Yang, J. Am. Chem. Soc. , 2015, 137 , 14129–14135 CrossRef CAS PubMed .
- S. He, Y. Chen, Z. Zhang, B. Ni, W. He and X. Wang, Chem. Sci. , 2016, 7 , 7101–7105 RSC .
- H. Xu, J. Gao, X. Qian, J. Wang, H. He, Y. Cui, Y. Yang, Z. Wang and G. Qian, J. Mater. Chem. A , 2016, 4 , 10900–10905 RSC .
- W. P. Lustig, S. Mukherjee, N. D. Rudd, A. V. Desai, J. Li and S. K. Ghosh, Chem. Soc. Rev. , 2017, 46 , 3242–3285 RSC .
- L. E. Kreno, K. Leong, O. K. Farha, M. Allendorf, R. P. Van Duyne and J. T. Hupp, Chem. Rev. , 2012, 112 , 1105–1125 CrossRef CAS PubMed .
- M. Zhao, Y. Wang, Q. Ma, Y. Huang, X. Zhang, J. Ping, Z. Zhang, Q. Lu, Y. Yu, H. Xu, Y. Zhao and H. Zhang, Adv. Mater. , 2015, 27 , 7372–7378 CrossRef CAS PubMed .
- H. S. Wang, H. L. Liu, K. Wang, Y. Ding, J. J. Xu, X. H. Xia and H. Y. Chen, Anal. Chem. , 2017, 89 , 11366–11371 CrossRef CAS PubMed .
- Y. Zhang, Y. Luo, J. Tian, A. M. Asiri, A. O. Al-Youbi and X. Sun, PLoS One , 2012, 7 , e30426, DOI: 10.1371/journal.pone.0030426 .
- Y. Y. Fan, Z. L. Mou, M. Wang, J. Li, J. Zhang, F. Q. Dang and Z. Q. Zhang, Anal. Chem. , 2018, 90 , 13708–13713 CrossRef CAS PubMed .
- C. He, K. Lu and W. Lin, J. Am. Chem. Soc. , 2014, 136 , 12253–12256 CrossRef CAS PubMed .
- Y. Peng, Y. Li, Y. Ban, H. Jin, W. Jiao, X. Liu and W. Yang, Science , 2014, 346 , 1356–1359, DOI: 10.1126/science.1254227 .
- Y. Peng, Y. Li, Y. Ban and W. Yang, Angew. Chem. , 2017, 129 , 9889–9893 CrossRef .
- S. Jiang, X. Shi, F. Sun and G. Zhu, Chem. – Asian J. , 2020, 3 , 1–9 Search PubMed .
- Y. Cheng, X. Wang, C. Jia, Y. Wang, L. Zhai, Q. Wang and D. Zhao, J. Membr. Sci. , 2017, 539 , 213–223 CrossRef CAS .
- M. Shete, P. Kumar, J. E. Bachman, X. Ma, Z. P. Smith, W. Xu, K. A. Mkhoyan, J. R. Long and M. Tsapatsis, J. Membr. Sci. , 2018, 549 , 312–320 CrossRef CAS .
- Z. Kang, Y. Peng, Z. Hu, Y. Qian, C. Chi, L. Y. Yeo, L. Tee and D. Zhao, J. Mater. Chem. A , 2015, 3 , 20801–20810 RSC .
- Y. Yang, K. Goh, R. Wang and T. H. Bae, Chem. Commun. , 2017, 53 , 4254–4257 RSC .
- H. L. Liu, Y. J. Chang, T. Fan and Z. Y. Gu, Chem. Commun. , 2016, 52 , 12984–12987 RSC .
- M. H. Pham, G. T. Vuong, F. G. Fontaine and T. O. Do, Cryst. Growth Des. , 2012, 12 , 3091–3095 CrossRef CAS .
- R. Chen, J. Yao, Q. Gu, S. Smeets, C. Baerlocher, H. Gu, D. Zhu, W. Morris, O. M. Yaghi and H. Wang, Chem. Commun. , 2013, 49 , 9500–9502 RSC .
- R. Kumar, K. Jayaramulu, T. K. Maji and C. N. R. Rao, Dalton Trans. , 2014, 43 , 7383–7386 RSC .
- J. Guo, Y. Zhang, Y. Zhu, C. Long, M. Zhao, M. He, X. Zhang, J. Lv, B. Han and Z. Tang, Angew. Chem., Int. Ed. , 2018, 57 , 6873–6877 CrossRef CAS PubMed .
- V. Galstyan, M. Bhandari, V. Sberveglieri, G. Sberveglieri and E. Comini, Chemosensors , 2018, 6 , 16 CrossRef .
- M. F. Baran, H. Acay and C. Keskin, Global Challenges , 2020, 4 , 1900104 CrossRef PubMed .
- G. Maduraiveeran, M. Sasidharan and W. Jin, Prog. Mater. Sci. , 2019, 106 , 100574 CrossRef CAS .
- J. Wu, X. Wang, Q. Wang, Z. Lou, S. Li, Y. Zhu, L. Qin and H. Wei, Chem. Soc. Rev. , 2019, 48 , 1004–1076 RSC .
- H. Bin Wu, J. S. Chen, H. H. Hng and X. Wen (David) Lou, Nanoscale , 2012, 4 , 2526 RSC .
- W. Zhang, J. Ai, Y. Lei, Y. Li, C. Lai and J. Xie, Solid State Ionics , 2020, 344 , 115132 CrossRef CAS .
- J. Ying, C. Wan, C. Jiang and Y. Li, J. Power Sources , 2001, 99 , 78–84 CrossRef CAS .
- B. Qu, L. Hu, Q. Li, Y. Wang, L. Chen and T. Wang, ACS Appl. Mater. Interfaces , 2014, 6 , 731–736 CrossRef CAS .
- P.-F. Wang, Y. You, Y.-X. Yin and Y.-G. Guo, Adv. Energy Mater. , 2018, 8 , 1701912 CrossRef .
- Y. Fang, X.-Y. Yu and X. W. (David) Lou, Matter , 2019, 1 , 90–114 CrossRef .
- T. Wang, D. Su, D. Shanmukaraj, T. Rojo, M. Armand and G. Wang, Electrochem. Energy Rev. , 2018, 1 , 200–237 CrossRef CAS .
- Q. Liu, Z. Hu, M. Chen, C. Zou, H. Jin, S. Wang, S. Chou and S. Dou, Small , 2019, 15 , 1805381 CrossRef PubMed .
- Y. Huang, Y. Zheng, X. Li, F. Adams, W. Luo, Y. Huang and L. Hu, ACS Energy Lett. , 2018, 3 , 1604–1612 CrossRef CAS .
- J. Ye, G. Xia, Z. Zheng and C. Hu, Int. J. Hydrogen Energy , 2020, 45 , 9969–9978 CrossRef CAS .
- F. Zhang, J. Zhu, D. Zhang, U. Schwingenschlögl and H. N. Alshareef, Nano Lett. , 2017, 17 , 1302–1311 CrossRef CAS PubMed .
- L. Liang, Y. Xu, C. Wang, L. Wen, Y. Fang, Y. Mi, M. Zhou, H. Zhao and Y. Lei, Energy Environ. Sci. , 2015, 8 , 2954–2962 RSC .
- L. Shen, Q. Che, H. Li and X. Zhang, Adv. Funct. Mater. , 2014, 24 , 2630–2637 CrossRef CAS .
- D. Kundu, B. D. Adams, V. Duffort, S. H. Vajargah and L. F. Nazar, Nat. Energy , 2016, 1 , 16119 CrossRef CAS .
- J. Ha, Y. Kim and J. Choi, ChemSusChem , 2020, 13 , 419–425 CrossRef CAS PubMed .
- Z. Kang, C. Wu, L. Dong, W. Liu, J. Mou, J. Zhang, Z. Chang, B. Jiang, G. Wang, F. Kang and C. Xu, ACS Sustainable Chem. Eng. , 2019, 7 , 3364–3371 CrossRef CAS .
- S. Lu, H. Wang, J. Zhou, X. Wu and W. Qin, Nanoscale , 2017, 9 , 1184–1192 RSC .
- J.-W. Lee, H.-S. Shin, C.-W. Lee and K.-N. Jung, Nanoscale Res. Lett. , 2016, 11 , 45 CrossRef PubMed .
- J. Han, J. Qin, L. Guo, K. Qin, N. Zhao, C. Shi, E. Liu, F. He, L. Ma and C. He, Appl. Surf. Sci. , 2018, 427 , 670–679 CrossRef CAS .
- Z. Zhang, X. Zhao and J. Li, ChemNanoMat , 2016, 2 , 196–200 CrossRef CAS .
- Y. Xiong, J. Qian, Y. Cao, X. Ai and H. Yang, ACS Appl. Mater. Interfaces , 2016, 8 , 16684–16689 CrossRef CAS PubMed .
- X. Wang, K. Cao, Y. Wang and L. Jiao, Small , 2017, 13 , 1700873 CrossRef PubMed .
- X. Li, X. Hao, A. Abudula and G. Guan, J. Mater. Chem. A , 2016, 4 , 11973–12000 RSC .
- Y. Yan, B. Y. Xia, B. Zhao and X. Wang, J. Mater. Chem. A , 2016, 4 , 17587–17603 RSC .
- I. Roger, M. A. Shipman and M. D. Symes, Nat. Rev. Chem. , 2017, 1 , 0003 CrossRef CAS .
- X. Zou and Y. Zhang, Chem. Soc. Rev. , 2015, 44 , 5148–5180 RSC .
- X. Yang, H. Li, A.-Y. Lu, S. Min, Z. Idriss, M. N. Hedhili, K.-W. Huang, H. Idriss and L.-J. Li, Nano Energy , 2016, 25 , 42–50 CrossRef CAS .
- A. G. Tamirat, J. Rick, A. A. Dubale, W.-N. Su and B.-J. Hwang, Nanoscale Horiz. , 2016, 1 , 243–267 RSC .
- Y. Yang, S. Niu, D. Han, T. Liu, G. Wang and Y. Li, Adv. Energy Mater. , 2017, 7 , 1700555 CrossRef .
- N. Nasori, T. Dai, X. Jia, A. Rubiyanto, D. Cao, S. Qu, Z. Wang, Z. Wang and Y. Lei, J. Semicond. , 2019, 40 , 052701 CrossRef CAS .
- Z. Yu, H. Liu, M. Zhu, Y. Li and W. Li, Small , 2019, 1903378 Search PubMed .
- V. Sharma, M. Prasad, P. Ilaiyaraja, C. Sudakar and S. Jadkar, Int. J. Hydrogen Energy , 2019, 44 , 11459–11471 CrossRef CAS .
- X. Li, C. Gao, H. Duan, B. Lu, Y. Wang, L. Chen, Z. Zhang, X. Pan and E. Xie, Small , 2013, 9 , 2005–2011 CrossRef CAS PubMed .
- M. Tayebi and B.-K. Lee, Renewable Sustainable Energy Rev. , 2019, 111 , 332–343 CrossRef CAS .
- J. Guo, Y. Li, B. Jiang, H. Gao, T. Wang, P. Sun, F. Liu, X. Yan, X. Liang, Y. Gao, J. Zhao and G. Lu, Sens. Actuators, B , 2020, 310 , 127780 CrossRef CAS .
- X. Chen and S. S. Mao, Chem. Rev. , 2007, 107 , 2891–2959 CrossRef CAS PubMed .
- K. Wetchakun, T. Samerjai, N. Tamaekong, C. Liewhiran, C. Siriwong, V. Kruefu, A. Wisitsoraat, A. Tuantranont and S. Phanichphant, Sens. Actuators, B , 2011, 160 , 580–591 CrossRef CAS .
- J. M. George, A. Antony and B. Mathew, Microchim. Acta , 2018, 185 , 358 CrossRef PubMed .
- A.-N. Kawde, M. Aziz, N. Baig and Y. Temerk, J. Electroanal. Chem. , 2015, 740 , 68–74 CrossRef CAS .
- R. Narayanan and M. A. El-Sayed, Nano Lett. , 2004, 4 , 1343–1348 CrossRef CAS .
- X. Xie, Y. Li, Z.-Q. Liu, M. Haruta and W. Shen, Nature , 2009, 458 , 746–749 CrossRef CAS PubMed .
- K. M. Bratlie, H. Lee, K. Komvopoulos, P. Yang and G. A. Somorjai, Nano Lett. , 2007, 7 , 3097–3101 CrossRef CAS PubMed .
- Z. Sun, T. Liao, L. Sheng, L. Kou, J. H. Kim and S. X. Dou, Chem. – Eur. J. , 2016, 22 , 11357–11364 CrossRef CAS PubMed .
- Q. Liang, X. Zou, H. Chen, M. Fan and G.-D. Li, Sens. Actuators, B , 2020, 304 , 127241 CrossRef CAS .
- S. Ozkan, N. T. Nguyen, I. Hwang, A. Mazare and P. Schmuki, Small , 2017, 13 , 1603821 CrossRef PubMed .
- S. Cheon, W. W. Lee, W. Il Park, J.-Y. Jung, J.-H. Choi, D.-G. Choi, S. Jeon, J. Jeong and J. Lee, J. Ind. Eng. Chem. , 2020, 81 , 385–392 CrossRef CAS .
- G. A. Cerrón-Calle, A. J. Aranda-Aguirre, C. Luyo, S. Garcia-Segura and H. Alarcón, Chemosphere , 2019, 219 , 296–304 CrossRef PubMed .
- X. Feng, Y. Huang, C. Li, X. Chen, S. Zhou, X. Gao and C. Chen, Chem. Eng. J. , 2019, 368 , 51–60 CrossRef CAS .
- L. Peng, P. Xiong, L. Ma, Y. Yuan, Y. Zhu, D. Chen, X. Luo, J. Lu, K. Amine and G. Yu, Nat. Commun. , 2017, 8 , 15139 CrossRef PubMed .
- K. Xu, S. Ma, Y. Shen, Q. Ren, J. Yang, X. Chen and J. Hu, Chem. Eng. J. , 2019, 369 , 363–369 CrossRef CAS .
- Y. Xie, C. Chen, X. Lu, F. Luo, C. Wang, A. Alsaedi and T. Hayat, J. Hazard. Mater. , 2019, 379 , 120786 CrossRef CAS PubMed .
- S. M. Saleh, Spectrochim. Acta, Part A , 2019, 211 , 141–147 CrossRef CAS PubMed .
- M. Guzman, M. Estrada, S. Miridonov and A. Simakov, Microporous Mesoporous Mater. , 2019, 278 , 241–250 CrossRef CAS .
- X. Zhang, W. Lan, J. Xu, Y. Luo, J. Pan, C. Liao, L. Yang, W. Tan and X. Huang, Sens. Actuators, B , 2019, 289 , 144–152 CrossRef CAS .
- M. Wang, Y. Huang, Y. Zhu, N. Zhang, J. Zhang, X. Qin and H. Zhang, Electrochim. Acta , 2020, 335 , 135694 CrossRef CAS .
- J. Feng and Y. Yin, Adv. Mater. , 2019, 31 , 1802349 CrossRef PubMed .
- Z. Wang, L. Zhou and X. W. David Lou, Adv. Mater. , 2012, 24 , 1903–1911 CrossRef CAS PubMed .
- X. W. Lou, Y. Wang, C. Yuan, J. Y. Lee and L. A. Archer, Adv. Mater. , 2006, 18 , 2325–2329 CrossRef CAS .
- H. Tianou, W. Wang, X. Yang, Z. Cao, Q. Kuang, Z. Wang, Z. Shan, M. Jin and Y. Yin, Nat. Commun. , 2017, 8 , 1261 CrossRef PubMed .
- S. Zhou, M. Chen, Q. Lu, J. Hu, H. Wang, K. Li, K. Li, J. Zhang, Z. Zhu and Q. Liu, Mater. Lett. , 2019, 247 , 15–18 CrossRef CAS .
- W. Xin, T. Gao, W. Zhang, T. Hu, X. Sun and G. Zhou, J. Alloys Compd. , 2019, 784 , 157–164 CrossRef CAS .
- D. Zhang, Z. Yang, Z. Wu and G. Dong, Sens. Actuators, B , 2019, 283 , 42–51 CrossRef CAS .
- T. Zhang, J. Zheng, Z. Liang, B. Zhao, H. Zeng, W. Guo, L. Zhao, Y. Sun, I. Abdulhalim and L. Jiang, Electrochim. Acta , 2019, 306 , 151–158 CrossRef CAS .
- B. Dong, W. Li, X. Huang, Z. Ali, T. Zhang, Z. Yang and Y. Hou, Nano Energy , 2019, 55 , 37–41 CrossRef CAS .
- R. Ghosh Chaudhuri and S. Paria, Chem. Rev. , 2012, 112 , 2373–2433 CrossRef CAS PubMed .
- W. Schärtl, Nanoscale , 2010, 2 , 829 RSC .
- L. León Félix, J. A. H. Coaquira, M. A. R. Martínez, G. F. Goya, J. Mantilla, M. H. Sousa, L. D. L. S. Valladares, C. H. W. Barnes and P. C. Morais, Sci. Rep. , 2017, 7 , 41732 CrossRef PubMed .
- S. Wei, Q. Wang, J. Zhu, L. Sun, H. Lin and Z. Guo, Nanoscale , 2011, 3 , 4474 RSC .
- F. Forato, S. Talebzadeh, N. Rousseau, J.-Y. Mevellec, B. Bujoli, D. A. Knight, C. Queffélec and B. Humbert, Phys. Chem. Chem. Phys. , 2019, 21 , 3066–3072 RSC .
- H. Mi, X. Zhang, S. An, X. Ye and S. Yang, Electrochem. Commun. , 2007, 9 , 2859–2862 CrossRef CAS .
- R. M. Abdel Hameed, New J. Chem. , 2018, 42 , 2658–2668 RSC .
- M.-Y. Kuo, C.-F. Hsiao, Y.-H. Chiu, T.-H. Lai, M.-J. Fang, J.-Y. Wu, J.-W. Chen, C.-L. Wu, K.-H. Wei, H.-C. Lin and Y.-J. Hsu, Appl. Catal., B , 2019, 242 , 499–506 CrossRef CAS .
- H. S. Choi, J. H. Park, J. W. Bae, J. H. Lee and T. S. Chang, Catal. Commun. , 2019, 123 , 86–90 CrossRef CAS .
- K. Awazu, M. Fujimaki, C. Rockstuhl, J. Tominaga, H. Murakami, Y. Ohki, N. Yoshida and T. Watanabe, J. Am. Chem. Soc. , 2008, 130 , 1676–1680 CrossRef CAS PubMed .
- S. V. Salihov, Y. A. Ivanenkov, S. P. Krechetov, M. S. Veselov, N. V. Sviridenkova, A. G. Savchenko, N. L. Klyachko, Y. I. Golovin, N. V. Chufarova, E. K. Beloglazkina and A. G. Majouga, J. Magn. Magn. Mater. , 2015, 394 , 173–178 CrossRef CAS .
- H. Wang, Q. Mu, R. Revia, K. Wang, B. Tian, G. Lin, W. Lee, Y.-K. Hong and M. Zhang, J. Controlled Release , 2018, 289 , 70–78 CrossRef CAS PubMed .
- K. C. Kwon, J. H. Ryu, J.-H. Lee, E. J. Lee, I. C. Kwon, K. Kim and J. Lee, Adv. Mater. , 2014, 26 , 6436–6441 CrossRef CAS PubMed .
- Y.-K. Kim, H.-K. Na, S. Kim, H. Jang, S.-J. Chang and D.-H. Min, Small , 2015, 11 , 2527–2535 CrossRef CAS PubMed .
- A. Seth, H. Gholami Derami, P. Gupta, Z. Wang, P. Rathi, R. Gupta, T. Cao, J. J. Morrissey and S. Singamaneni, ACS Appl. Mater. Interfaces , 2020, 12 , 42499–42510 CrossRef CAS PubMed .
- Y. Xiang, X. Peng, X. Kong, Z. Tang and H. Quan, Colloids Surf., A , 2020, 594 , 124652 CrossRef CAS .
- Q. Lv, H. Min, D. Duan, W. Fang, G. Pan, A. Shen, Q. Wang, G. Nie and J. Hu, Adv. Healthcare Mater. , 2018, 8 , 1801257 CrossRef PubMed .
- L. Li, Y. Lu, C. Jiang, Y. Zhu, X. Yang, X. Hu, Z. Lin, Y. Zhang, M. Peng, H. Xia and C. Mao, Adv. Funct. Mater. , 2018, 28 , 1704623 CrossRef PubMed .
- T. Liu, X. Li, J. Wang, P. Zhang, X. Huang, Z. Zhang, D.-S. Guo and X. Yang, J. Mater. Chem. B , 2020, 8 , 5483–5490 RSC .
- K.-C. Ho and L.-Y. Lin, J. Mater. Chem. A , 2019, 7 , 3516–3530 RSC .
- Y. Bai, R. Zhang, X. Ye, Z. Zhu, H. Xie, B. Shen, D. Cai, B. Liu, C. Zhang, Z. Jia, S. Zhang, X. Li and F. Wei, Nat. Nanotechnol. , 2018, 13 , 589–595 CrossRef CAS PubMed .
- F. Yang, X. Wang, D. Zhang, J. Yang, D. Luo, Z. Xu, J. Wei, J.-Q. Wang, Z. Xu, F. Peng, X. Li, R. Li, Y. Li, M. Li, X. Bai, F. Ding and Y. Li, Nature , 2014, 510 , 522–524 CrossRef CAS PubMed .
- J. Liu, J. Lu, X. Lin, Y. Tang, Y. Liu, T. Wang and H. Zhu, Comput. Mater. Sci. , 2017, 129 , 290–294 CrossRef CAS .
- C. R. Vandenabeele and S. Lucas, Mater. Sci. Eng., R , 2020, 139 , 100521 CrossRef .
- Y. He, W. Chen, X. Li, Z. Zhang, J. Fu, C. Zhao and E. Xie, ACS Nano , 2013, 7 , 174–182 CrossRef CAS PubMed .
- C. Li and G. Shi, Nanoscale , 2012, 4 , 5549 RSC .
- P. Tucci, G. Porta, M. Agostini, D. Dinsdale, I. Iavicoli, K. Cain, A. Finazzi-Agró, G. Melino and A. Willis, Cell Death Dis. , 2013, 4 , e549–e549 CrossRef CAS PubMed .
- T. G. Smijs and S. Pavel, Nanotechnol., Sci. Appl. , 2011, 4 , 95–112 CrossRef CAS PubMed .
- W. J. Stark, P. R. Stoessel, W. Wohlleben and A. Hafner, Chem. Soc. Rev. , 2015, 44 , 5793–5805 RSC .
Open Access is an initiative that aims to make scientific research freely available to all. To date our community has made over 100 million downloads. It’s based on principles of collaboration, unobstructed discovery, and, most importantly, scientific progression. As PhD students, we found it difficult to access the research we needed, so we decided to create a new Open Access publisher that levels the playing field for scientists across the world. How? By making research easy to access, and puts the academic needs of the researchers before the business interests of publishers.
We are a community of more than 103,000 authors and editors from 3,291 institutions spanning 160 countries, including Nobel Prize winners and some of the world’s most-cited researchers. Publishing on IntechOpen allows authors to earn citations and find new collaborators, meaning more people see your work not only from your own field of study, but from other related fields too.
Brief introduction to this section that descibes Open Access especially from an IntechOpen perspective
Want to get in touch? Contact our London head office or media team here
Our team is growing all the time, so we’re always on the lookout for smart people who want to help us reshape the world of scientific publishing.
Home > Books > Green Chemistry for Environmental Sustainability - Prevention-Assurance-Sustainability (P-A-S) Approach
Green Synthesis of Nanoparticles: A Biological Approach
Submitted: 29 May 2023 Reviewed: 05 June 2023 Published: 11 August 2023
DOI: 10.5772/intechopen.1002203
Cite this chapter
There are two ways to cite this chapter:
From the Edited Volume
Green Chemistry for Environmental Sustainability - Prevention-Assurance-Sustainability (P-A-S) Approach
Kinjal J. Shah
To purchase hard copies of this book, please contact the representative in India: CBS Publishers & Distributors Pvt. Ltd. www.cbspd.com | [email protected]
Chapter metrics overview
756 Chapter Downloads
Impact of this chapter
Total Chapter Downloads on intechopen.com

Total Chapter Views on intechopen.com
Nanoparticles are often associated with their small size and numerous applications. However, the synthesis process is equally important as it determines the size and properties of the nanoparticles. While traditional nanoparticle synthesis methods require the use of hazardous chemicals and high-energy consumption, green synthesis offers a sustainable, cost-effective, and environmentally friendly alternative. This approach utilizes natural resources and biologically active compounds that can act as reducing, stabilizing, or capping agents in the one-step synthesis of nanoparticles. Green synthesis offers numerous advantages, including the development of processes with minimal environmental impact and improved safety for nanoparticle synthesis. Overall, the synthesis of nanoparticles using green chemistry is a promising approach for sustainable and efficient production. This chapter provides a general overview of nanoparticles, their applications, and green synthesis, and highlights the various biological resources used in these processes and the factors affecting their synthesis.
- green synthesis
- nanoparticles
- plant extract
- microorganisms
- phytochemicals
Author Information
Rafael álvarez-chimal *.
- Laboratory 113 Synthesis of Magnetic Nanomaterials, Condensed Matter Department, Institute of Physic, National Autonomous University of Mexico, Ciudad Universitaria, Mexico City, Coyoacán, Mexico
Jesús Ángel Arenas-Alatorre
*Address all correspondence to: [email protected]
1. Introduction
Nanoparticles are small particles with sizes ranging from 1 to 100 nanometers. These materials have gained importance and interest in recent years owing to their large number of applications, because the matter at this scale presents a more compact arrangement of atoms and molecules, generating phenomena and acquiring or enhancing mechanical [ 1 ], electrical [ 2 ], magnetic [ 3 ], optical [ 4 ], catalytic [ 5 ], and antibacterial [ 6 , 7 ] properties that are completely different from those of their macroscopic counterparts [ 8 ]. They can be classified based on their composition, shape, and size. The most common types of nanoparticles are metals, metal oxides, carbon-based, and quantum dots. Owing to their unique sizes and properties, nanoparticles have attracted significant attention in various fields including medicine, electronics, energy, and environmental science [ 9 , 10 ]. By reducing their size, nanoparticles can have a higher surface-to-volume ratio, enabling a greater number of atoms or molecules per volume, which means that less material is needed to obtain the same activity and exhibit other properties ( Figure 1 ) [ 11 ].
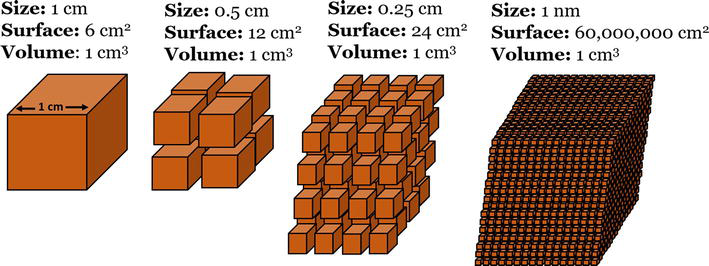
Surface-to-volume ratio of nanoparticles compared with that of bulk materials.
Nanoparticles have many potential benefits for the environment. For example, nanoparticles can be used to improve the efficiency of water treatment, air filtration, and soil remediation; reduce pollution, and develop new types of renewable energy technologies [ 12 ]. In medicine, nanoparticles have shown potential for drug delivery, imaging, and cancer therapy. They can be functionalized with targeting moieties, making them capable of selectively targeting cancer cells, while sparing normal cells. Additionally, nanoparticles can enhance the efficacy of chemotherapy by improving drug delivery to the tumor site and reducing systemic toxicity [ 13 ]. In electronics, nanoparticles are used to fabricate high-performance devices such as sensors, transistors, and solar cells [ 14 ]. Nanoparticles have potential applications in fuel cells, hydrogen storage, and catalysis [ 15 ].
However, it is also important to address the environmental impact of the nanoparticles. Some studies have shown that nanoparticles can harm plants, animals, and humans, but it depends on many factors, such as concentration, size, and time of exposure [ 16 , 17 ]. Nanoparticles can easily be released into the environment through various sources, such as industrial emissions, consumer products, and medical procedures. Once released into the environment, nanoparticles can be difficult to control and monitor. There is potential for long-term accumulation. Nanoparticles can accumulate in the environment, and they may be able to persist for long periods. This raises concerns about the potential for nanoparticles to cause long-term harm to the environment and human health [ 17 , 18 ]. However, one of the alternatives for reducing their environmental impact is to control the synthesis process.
There are many methods for synthesizing nanoparticles, including physical, chemical, and biological processes [ 19 ]. Green synthesis, which refers to the eco-friendly and sustainable production of nanoparticles without the use of hazardous chemicals or toxic solvents, has gained attention in recent years within biological processes. Natural sources, such as plants and microorganisms, are popular green synthesis approaches [ 20 ]. This method has several advantages over traditional synthesis methods, including low cost, scalability, and reduction of hazardous waste. Moreover, green synthesis can produce nanoparticles with unique shapes, sizes, and surface properties tailored for specific applications [ 21 ]. The biological sources used for the green synthesis of nanoparticles contain biologically active compounds, such as enzymes, proteins, polyphenols, flavonoids, and terpenoids, which can act as catalyzing, reducing, stabilizing, or capping agents for one-step synthesis [ 20 , 21 ].
In summary, this chapter provides a general overview of nanoparticles, their properties and applications, and how green synthesis is used to synthesize them. This chapter also discusses the different biological resources used for green synthesis, the factors that participate, and the mechanisms involved in their production.
2. Traditional nanoparticle synthesis methods
Chemical reduction: This method involves the reduction of metal ions in solution using chemical reagents such as sodium borohydride or sodium hydroxide to form nanoparticles [ 22 ].
Coprecipitation: Synthesis involves mixing two or more solutions containing metal ions. When the solutions are mixed, metal ions precipitate out of the solution and form nanoparticles [ 23 ].
Sol-gel: The process requires mixing a metal salt with a solvent and gelling agent. The solvent is evaporated leaving behind the gel. The gel is then heated, causing it to solidify and form nanoparticles [ 24 ].
Microemulsion: This method needs surfactants, water-soluble compounds, and oil-soluble compounds. The mixture forms small droplets that contain the metal ions. When droplets are heated, metal ions precipitate out of the solution and form nanoparticles [ 25 ].
Solvothermal/hydrothermal synthesis: This reaction involves heating a solution of metal ions in water or an organic solvent under high pressure. High pressure and temperature cause metal ions to precipitate out of the solution and form nanoparticles [ 26 ].
Sonochemical/electrochemical synthesis: This process uses ultrasound or an electrical current to break down metal salts into nanoparticles [ 27 ].
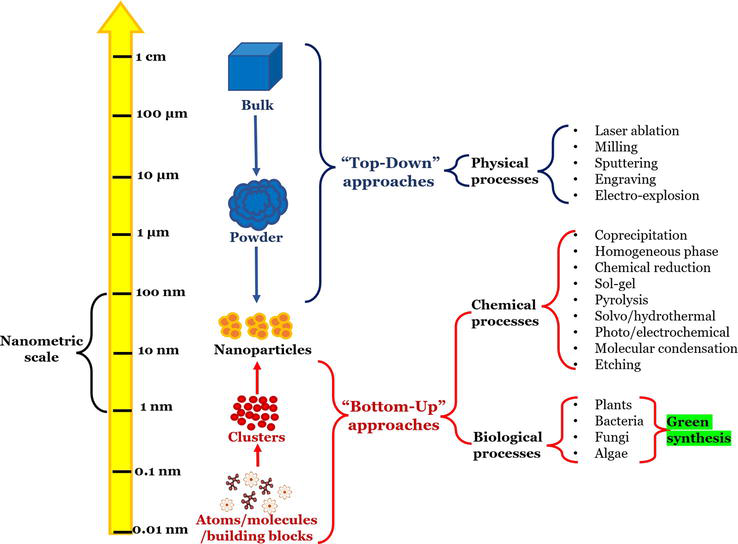
Nanometric scale and different approaches to nanoparticle synthesis.
In addition, there are physical processes, such as laser ablation, milling, and sputtering, where the material is reduced to nanoparticles by the mechanical action of the equipment used [ 28 ].
The choice of method depends on the type of nanoparticles being synthesized, the desired size and shape, and the availability of equipment and reagents.
2.1 Environmental limitations in nanoparticle synthesis
Traditional methods for synthesizing nanoparticles have several limitations.
Using organic reagents can harm the environment, humans, and animals, causing illnesses, such as liver damage [ 18 ]. In addition, wastewater generated from nanoparticle synthesis can contain harmful chemicals [ 29 ].
The low yield is another disadvantage: only a small percentage of the starting materials is converted into nanoparticles, generating raw material waste. The high cost of the starting materials, equipment, labor required, long-time synthesis, and the inability to control the size and shape can limit their applications [ 30 , 31 ].
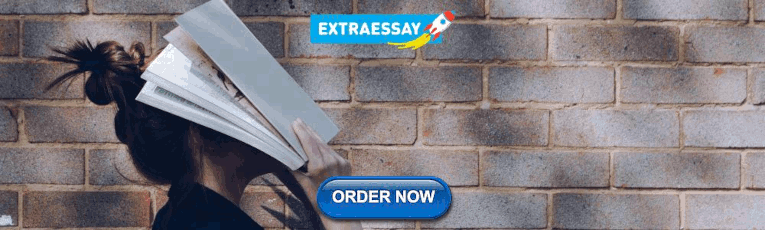
2.2 Strategies to overcome barriers to nanoparticle synthesis
Several strategies can be used to overcome the disadvantages of nanoparticle synthesis, such as the use of environmentally friendly solvents, reagents, and processes. Using water, ionic liquids, and supercritical fluids are examples of eco-friendly solvents [ 21 , 32 ] or we can even perform solvent-free synthesis, eliminating the need for hazardous chemicals and reducing the environmental impact of nanoparticle synthesis [ 33 ].
Many nanoparticle synthesis methods are not scalable, which limits their application. Therefore, it is necessary to develop cost-effective and efficient processes to obtain large quantities of nanoparticles [ 8 ].
Multipurpose nanoparticles can be used to improve their performance in a variety of applications and fields. For example, biocompatible nanoparticles are used in biomedicine or as stable nanoparticles for long-term applications [ 34 ].
The characterization of nanoparticles is important for understanding their size, shape, surface properties, and chemical composition. This information can be used to understand how nanoparticles interact with their environment and ensure they are safe [ 35 ].
Strategies to overcome these barriers in nanoparticle synthesis are still under study to develop more innovative, efficient, cost-effective, and environmentally friendly methods.
3. Green synthesis of nanoparticles: an overview
Green synthesis aims to promote innovative chemical technologies to reduce or eliminate the use and production of hazardous substances in the design, manufacture, and use of chemical products. This involves minimizing or, if possible, eliminating the pollution produced in the synthesis processes, avoiding the consumption and wastage of nonrenewable raw materials, using hazardous or polluting materials in product manufacturing, and reducing the synthesis time. Paul J. Anastas, considered the father of green chemistry, defined it as “a work philosophy that involves the use of alternative tools and pathways to prevent pollution,” referring to both the design of the synthetic strategy and the treatment of possible secondary products originating from that route [ 36 , 37 ].
Two approaches can be used to generate nanoparticles [ 37 , 38 ] ( Figure 2 ).
“Top-down” approach: In which nanoparticles are produced using physical techniques such as grinding or abrasion of a material.
Chemical synthesis: The method of producing molecules or particles by the reaction of substances used as raw materials.
Self-assembly: A technique in which atoms or molecules self-order through physical and/or chemical interactions.
Positional assembly: The atoms, molecules, and aggregates are deliberately manipulated and positioned individually. However, this method is extremely laborious and unsuitable for industrial applications.
The “bottom-up” approach is preferred over the “top-down” approach because specialized equipment is not required and the time to obtain nanoparticles is shorter. Green synthesis is gaining relevance in producing nanoparticles within the “bottom-up” approach [ 37 ].
The use of plant species, algae, or microorganisms such as bacteria or fungi is one of the most commonly used resources for this procedure. Various compounds from plants or microorganisms, including terpenes, polyphenols, alkaloids, carbohydrates, proteins, and genetic materials, play an important role in the synthesis of nanoparticles by acting together [ 39 , 40 ].
In addition to the biological resources used to perform the synthesis (plants, algae, or microorganisms), other factors influence the shape and size of nanoparticles, such as the concentration of the metal ion, pH, reaction time, and temperature [ 39 , 41 ].
Initial phase: Obtaining the reaction medium, which is the aqueous extract of one or several parts of the plant species or the culture media for the growth of microorganisms, in addition to the precursor salt, which is the source of metal ions.
Activation phase: Chemical reduction of metal ions and generation of nucleation centers occur where nanoparticles emerge and grow.
Growth phase: Small adjacent nanoparticles spontaneously fuse into larger particles, forming aggregates, which are influenced by factors such as temperature, concentration, and type of compounds, pH, and reaction time.
Termination phase: The final shape of the nanoparticles is determined, and the compounds that participate in the reaction help stabilize and enhance their properties.

Phases involved in the green synthesis of nanoparticles.
3.1 Biological resources for the green synthesis of nanoparticles
As stated previously, nanoparticles have attracted attention in the fields of biology, medicine, and electronics in recent years, owing to their remarkable applications ( Figure 4 ). Numerous nanoparticle synthesis techniques have been developed; however, these may involve the use of toxic compounds and high-energy physical processes. An alternative is the use of biological methods to circumvent these obstacles. Bacteria, fungi, algae, and plant species are some of the most commonly used biological resources for the green synthesis of nanoparticles ( Figure 4 ). This biological approach has provided a method that is reliable, straightforward, benign, and environmentally beneficial [ 40 , 42 ].
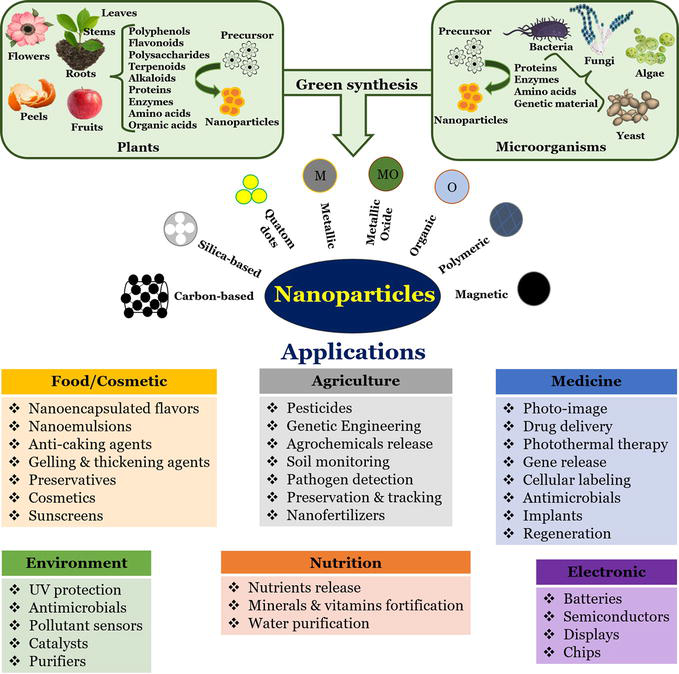
Biological resources and compounds used for the green synthesis of nanoparticles and some of their applications [ 9 ].
3.1.1 Bacteria
Nanoparticle synthesis using bacteria is performed both extracellularly and intracellularly [ 38 ].
Intracellular: The synthesis is carried out inside the living microorganism, using its growth conditions to favor synthesis, known as “nanoparticle micro-factories.” To recover nanoparticles, bacteria must be destroyed [ 43 ].
Extracellular: The components released by the bacteria after lysis are used. The synthesis is performed by adding a metal salt precursor to the medium in which these components are located. Extracellular synthesis has the advantage of being faster because it does not require additional steps to recover nanoparticles from microorganisms [ 43 , 44 ].
Enzymes, such as reductases, which catalyze the reduction of metal ions into nanoparticles, participate in the synthesis. Even components of the genetic material participate in this process [ 45 , 46 ].
3.1.2 Fungi
Fungi contain active biomolecules, such as proteins or enzymes, that participate in nanoparticle synthesis, improving their yields and stability [ 47 ].
Some fungal species can synthesize nanoparticles using extracellular amino acids. For example, glutamic and aspartic acids on the surface of yeast or the reductase enzyme in the cytosol of fungi reduce metal ions to form nanoparticles. This is facilitated by the presence of hydroxyl groups in the mycelium, which donate electrons to the metal ion and reduce it to form nanoparticles. Aliphatic and aromatic amines or some proteins act as coating agents to stabilize them [ 48 , 49 ].
3.1.3 Algae
Algae are used in nanotechnology because of their low toxicity and their ability to bioaccumulate and reduce metals [ 50 ].
Nanoparticle synthesis can be intracellular, with the metal ion entering the alga, or extracellular, and involves compounds such as polysaccharides, proteins, and pigments that direct the reduction of metal ions and coat the newly formed nanoparticles. These particles are subsequently released from the cell in the form of colloids [ 51 ].
3.1.4 Plant species
The use of plants in nanoparticle synthesis is one of the most widely used methods because of its environmentally friendly nature, as it avoids the use of toxic or harmful substances. It is also one of the fastest and most economical methods because it involves fewer steps [ 39 , 40 ]. This makes it highly efficient in the nanoparticle production process compared to synthesis using microorganisms.
Plants contain several compounds (terpenes, flavonoids, polyphenols, alkaloids, proteins, etc.) that reduce metal ions and stabilize the resulting nanoparticles [ 52 ].
This type of synthesis can be performed using intracellular, extracellular, and phytochemical-mediated methods [ 53 ].
Intracellular: The synthesis is carried out inside the plant cell, and the nanoparticles are recovered by breaking down the structure, which is very similar to the intracellular method using microorganisms. Control of the growth factors of plant species is required so that they do not interfere with synthesis [ 54 ].
Extracellular: This method is the most commonly used because of its ease and speed. The process begins by obtaining a plant extract, which is generally water-based, to which a metal salt precursor is added. Owing to the action of the different compounds present in the extract, nanoparticles are generated and stabilized in a single step [ 54 , 55 ].
Phytochemically mediated: This is based on the extracellular method, but with the difference that isolated phytochemical compounds are used and other substances are added to stabilize the nanoparticles. There is greater control over the synthesis, but more components and steps are involved [ 53 ].
3.2 Factors involved in the green synthesis of nanoparticles
As in any synthesis process, reaction conditions, such as temperature, pH, and reaction time, play an important role in the shape, size, and yield of the synthesized nanoparticles [ 39 , 40 , 41 ] ( Figure 3 ).
Temperature: This is one of the most influential factors, as the shape (spherical, prismatic, flakes, triangular, octahedral, etc.), size, and synthesis depend on temperature. As the temperature increases, the reaction rate and the formation of nucleation centers increase, resulting in higher yields. Different temperatures promote different interactions between the reactants, giving rise to various shapes; the larger the temperature increase, the larger the size of the nanoparticles [ 56 , 57 ].
pH: This influences the nucleation centers, generating more centers at higher pH values. Another important influence of pH is that some nanoparticles can only be synthesized in acidic or alkaline media. For example, magnetic nanoparticles are synthesized at an alkaline pH, and metal oxide nanoparticles are generally synthesized at an acidic or neutral pH [ 58 ].
Time: This parameter plays an important role in defining the size of the nanoparticles. It has been observed that longer reaction times favor an increase in the size of the nanoparticles and higher yields, owing to the prolonged interaction time between reactants [ 59 ].
3.3 The mechanism involved in the green synthesis of nanoparticles
The plant extract or organism used for the synthesis is an important factor that influences the morphology and size of nanoparticles because different concentrations of metabolites or cellular components give rise to differences in the nanoparticles [ 40 , 60 ] ( Figure 5 ).
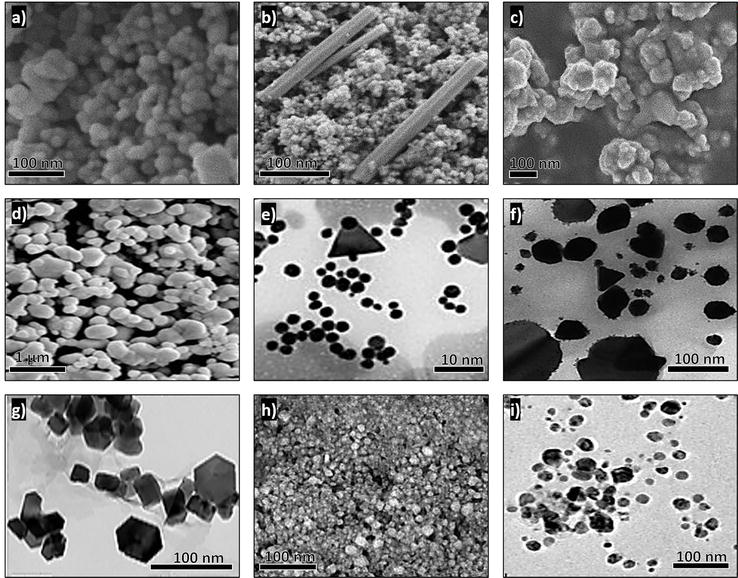
Green-synthesized nanoparticles. (a) Spherical ZnO nanoparticles using the leaves of Dysphania ambrosioides (plant). (b) Prismatic ZnO nanoparticles using the stems and leaves of Dysphania ambrosioides (plant). (c) Quasi-spherical Fe 3 O 4 nanoparticles using the leaves of Datura innoxia (plant). (d) Quasi-spherical Ag nanoparticles using stems of Aloe vera (plant) [ 61 ]. (e) Spherical and triangular Au nanoparticles using Lentinula edodes (fungus) [ 43 ]. (f) Irregular Ag and triangular Au nanoparticles using Ganoderma lucidum (fungus) [ 43 ]. (g) Hexagonal MgO nanoparticles using the flowers of Saussurea costus (plant) [ 62 ]. (h) Irregular Cu nanoparticles using Salmonella typhimurium (bacterium) [ 63 ]. (i) Quasi-spherical Ag nanoparticles using Dunaliella salina (alga) [ 64 ].
Proteins and enzymes facilitate the formation of nanoparticles from metal ions. Because of their high reducing activity, proteins and enzymes can attract metal ions to specific regions of a molecule responsible for reduction, facilitating the formation of nanoparticles; however, their chelating activity is not excessive. The amino acids of a protein can greatly influence the size, morphology, and quantity of nanoparticles generated, thus playing a very important role in determining their shape and yield. Removing a proton from amino acids or other molecules results in the formation of resonant structures capable of further oxidation. This process is accompanied by the active reduction of metal ions followed by the formation of nanoparticles [ 39 ].
Flavonoids are a large group of polyphenolic compounds that can actively chelate and reduce metal ions because they contain multiple functional groups capable of forming these structures. Structural transformations of flavonoids also generate protons that reduce metal ions to form nanoparticles; therefore, they are involved in the nucleation stage, their formation, and further aggregation. Saccharides can also play a role in nanoparticle formation. Monosaccharides, such as glucose, can act as reducing agents, as the aldehyde group of the sugar is oxidized to a carboxyl group through the addition of hydroxyl groups, which in turn leads to the reduction of metal ions and the synthesis of nanoparticles [ 39 ].
The mechanism of green synthesis of nanoparticles has been associated with the action of polyphenols, which act as ligands. Metal ions form coordination compounds, in which the fundamental structural unit is the central metal ion surrounded by coordinated groups arranged spatially at the corners of a regular tetrahedron. The aromatic hydroxyl groups in polyphenols bind to metal ions and form stable coordinated complexes. This system undergoes direct decomposition at high temperatures, releasing nanoparticles from the complex system [ 65 ].
Flavonoids, amino acids, proteins, terpenoids, tannins, and reducing sugars have hydroxyl groups that surround the metal ions to form complexes. After this process, the hydroxyl ions are oxidized to carbonyl groups, which stabilize the nanoparticles. Synthesis is favored if the participating molecules have at least two hydroxyl groups at the ortho- and para-positions [ 52 , 65 ].
Amino acids influence the size, morphology, and yield of nanoparticles generated [ 23 ], depending on the specific amino acids present in the extract and their concentration, along with the reaction conditions that give rise to nanoparticles with different shapes [ 65 ].
4. Confirming that the biological approach of nanoparticle synthesis is a green chemistry method
To corroborate that the processes of nanoparticle synthesis using biological resources are “green synthesis methods,” the 12 principles mentioned above are revisited [ 66 , 67 , 68 ] ( Table 1 ).
The 12 principles of green synthesis are fulfilled with the biological approach to produce nanoparticles.
Considering the above, the 12 principles of green synthesis are fulfilled using biological resources, such as plants, bacteria, fungi, and algae, to synthesize nanoparticles [ 69 , 70 , 71 ].
Finally, green synthesis of nanoparticles is a sustainable and environmentally friendly alternative to traditional methods of nanoparticle synthesis. Traditional methods often take long periods of time, use toxic chemicals and solvents, or generate waste products that can pollute the environment and pose health risks to humans and animals. In contrast, the green synthesis method uses renewable natural resources, such as plant extracts and microorganisms, which are less damaging and can be replenished over time. In addition, these methods are often more cost-effective and faster than traditional procedures because they do not require expensive chemicals or equipment and are considered one-step syntheses, which contribute to energy savings [ 72 ].
In furtherance of these advantages, green synthesis methods are still being developed to improve their efficiency and scalability, leading to the potential benefits of green synthesis of nanoparticles or even their application to the synthesis of other molecules as drugs or nutraceuticals.
5. Conclusion
Nanoparticles have emerged as a versatile and promising class of materials with unique properties that can be harnessed for various applications. The use of green synthesis utilizing natural resources and biologically active compounds to produce nanoparticles is an area of continuous research to improve processes, reduce environmental damage, and meet the increasing demand for the application of these nanostructures. Utilizing biological resources, the synthesis of nanoparticles is inexpensive, faster, and considered a one-step synthesis while preserving or even improving the physical and chemical properties of the nanoparticles. With the great potential of this method and the sustainable and efficient production of nanoparticles, different sizes and shapes can be obtained, which makes it a very attractive option not only for the synthesis of nanostructures, but also for the application of this technique in the synthesis of other compounds.
Acknowledgments
The authors acknowledge Dr. Samuel Tehuacanero Cuapa, Physicist. Roberto Hernández Reyes, and Arq. Diego Quiterio Vargas for their technical support.
Thanks to the Consejo Nacional de Humanidades, Ciencias y Tecnologías (CONAHCYT) for the scholarship granted to Rafael Álvarez-Chimal with the CVU number: 579637.
Funding was provided by the UNAM-DGAPA- PAPIIT project IN112422.
Conflict of interest
The authors declare no conflicts of interest.
- 1. Wu Q , Shou MW, Du ZY, Jun GH, Hui D. Mechanical properties of nanomaterials: A review. Nanotechnology Reviews. 2020; 9 (1):259-273. DOI: 10.1515/ntrev-2020-0021
- 2. Min SH, Lee TH, Lee S, Song JH, Lee GY, Zontar D, et al. Simulation of electrical conductivity for nanoparticles and nanotubes composite sensor according to geometrical properties of nanomaterials. Composites Part B: Engineering. 2019; 174 :107003. DOI: 10.1016/j.compositesb.2019.107003
- 3. Martínez-Mera I, Espinosa-Pesqueira ME, Pérez-Hernández R, Arenas-Alatorre J. Synthesis of magnetite (Fe 3 O 4 ) nanoparticles without surfactants at room temperature. Materials Letters. 2007; 61 (23-24):4447-4451. DOI: 10.1016/j.matlet.2007.02.018
- 4. Kelly KL, Coronado E, Zhao LL, Schatz GC. The optical properties of metal nanoparticles: The influence of size, shape, and dielectric environment. The Journal of Physical Chemistry. B. 2003; 107 (3):668-677. DOI: 10.1021/jp026731y
- 5. Zeng J, Zhang Q , Chen J, Xia Y. A comparison study of the catalytic properties of Au-based nanocages, nanoboxes, and nanoparticles. Nano Letters. 2010; 10 (1):30-35. DOI: 10.1021/nl903062e
- 6. Álvarez-Chimal R, García-Pérez VI, Álvarez-Pérez MA, Arenas-Alatorre JÁ. Green synthesis of ZnO nanoparticles using a Dysphania ambrosioides extract. Structural characterization and antibacterial properties. Materials Science and Engineering: C. 2021; 118 :111540. DOI: 10.1016/j.msec.2020.111540
- 7. Reyes-Carmona L, Camps E, Campos-González E, Mercado-Celis G, Cervantes-Garduño A, Pérez-Ibarra EA, et al. Antimicrobial evaluation of bismuth subsalicylate nanoparticles synthesized by laser ablation against clinical oral microorganisms. Optics and Laser Technology. 2023; 158 :108930. DOI: 10.1016/j.optlastec.2022.108930
- 8. Baig N, Kammakakam I, Falath W. Nanomaterials: A review of synthesis methods, properties, recent progress, and challenges. Materials Advances. 2021; 2 (6):1821-1871. DOI: 10.1039/D0MA00807A
- 9. Chaudhry N, Dwivedi S, Chaudhry V, Singh A, Saquib Q , Azam A, et al. Bio-inspired nanomaterials in agriculture and food: Current status, foreseen applications and challenges. Microbial Pathogenesis. 2018; 123 :196-200. DOI: 10.1016/j.micpath.2018.07.013
- 10. Kolahalam LA, Kasi Viswanath IV, Diwakar BS, Govindh B, Reddy V, Murthy YLN. Review on nanomaterials: Synthesis and applications. Materials Today: Proceedings. 2019; 18 :2182-2190. DOI: 10.1016/j.matpr.2019.07.371
- 11. Khan I, Saeed K, Khan I. Nanoparticles: Properties, applications and toxicities. Arabian Journal of Chemistry. 2019; 12 (7):908-931. DOI: 10.1016/j.arabjc.2017.05.011
- 12. Lu AH, Salabas EL, Schüth F. Magnetic nanoparticles: Synthesis, protection, functionalization, and application. Angewandte Chemie, International Edition. 2007; 46 (8):1222-1244. DOI: 10.1002/anie.200602866
- 13. Zhang L, Gu F, Chan J, Wang A, Langer R, Farokhzad O. Nanoparticles in medicine: Therapeutic applications and developments. Clinical Pharmacology and Therapeutics. 2008; 83 (5):761-769. DOI: 10.1038/sj.clpt.6100400
- 14. Gao J, Gu H, Xu B. Multifunctional magnetic nanoparticles: Design, synthesis, and biomedical applications. Accounts of Chemical Research. 2009; 42 (8):1097-1107. DOI: 10.1021/ar9000026
- 15. Singh R, Altaee A, Gautam S. Nanomaterials in the advancement of hydrogen energy storage. Heliyon. 2020; 6 (7):e04487. DOI: 10.1016/j.heliyon.2020.e04487
- 16. Yao D, Chen Z, Zhao K, Yang Q , Zhang W. Limitation and challenge faced to the researches on environmental risk of nanotechnology. Procedia Environmental Sciences. 2013; 18 :149-156. DOI: 10.1016/j.proenv.2013.04.020
- 17. Wilson N. Nanoparticles: Environmental problems or problem solvers? Bioscience. 2018; 68 (4):241-246. DOI: 10.1093/biosci/biy015
- 18. Ray PC, Yu H, Fu PP. Toxicity and environmental risks of nanomaterials: Challenges and future needs. Journal of Environmental Science and Health, Part C. 2009; 27 (1):1-35. DOI: 10.108010590500802708267/
- 19. Iravani S, Korbekandi H, Mirmohammadi SV, Zolfaghari B. Synthesis of silver nanoparticles: Chemical, physical and biological methods. Research in Pharmaceutical Sciences. 2014; 9 (6):385-406
- 20. Mustapha T, Misni N, Ithnin NR, Daskum AM, Unyah NZ. A review on plants and microorganisms mediated synthesis of silver nanoparticles, role of plants metabolites and applications. International Journal of Environmental Research and Public Health. 2022; 19 (2):674. DOI: 10.3390/ijerph19020674
- 21. Shafey AME. Green synthesis of metal and metal oxide nanoparticles from plant leaf extracts and their applications: A review. Green Processing and Synthesis. 2020; 9 (1):304-339. DOI: 10.1515/gps-2020-0031
- 22. Manikam VR, Cheong KY, Razak KA. Chemical reduction methods for synthesizing Ag and Al nanoparticles and their respective nanoalloys. Materials Science and Engineering B. 2011; 176 (3):187-203. DOI: 10.1016/j.mseb.2010.11.006
- 23. Nam NH, Luong NH. Nanoparticles: Synthesis and applications. In: Materials for Biomedical Engineering. Amsterdam, Netherlands: Elsevier; 2019. pp. 211-240. DOI: 10.1016/B978-0-08-102814-8.00008-1
- 24. Bokov D, Turki Jalil A, Chupradit S, Suksatan W, Javed Ansari M, Shewael IH, et al. Nanomaterial by sol-gel method: Synthesis and application. Advances in Materials Science and Engineering. 2021; 2021 :1-21. DOI: 10.1155/2021/5102014
- 25. Cid A. Synthesis of NPs by microemulsion method. In: Microemulsion - A Chemical Nanoreactor. London, UK: IntechOpen; 2018. DOI: 10.5772/intechopen.80633
- 26. Ndlwana L, Raleie N, Dimpe KM, Ogutu HF, Oseghe EO, Motsa MM, et al. Sustainable hydrothermal and solvothermal synthesis of advanced carbon materials in multidimensional applications: A review. Materials. 2021; 14 (17):5094. DOI: 10.3390/ma14175094
- 27. Ashassi-Sorkhabi H, Rezaei-moghadam B, Bagheri R, Abdoli L, Asghari E. Synthesis of Au nanoparticles by thermal, sonochemical and electrochemical methods: Optimization and characterization. Polymerase Chain Reaction. 2015; 3 (1):24-34. DOI: 10.22036/pcr.2015.7311
- 28. Krishnia L, Thakur P, Thakur A. Synthesis of Nanoparticles by Physical Route. Synthesis and Applications of Nanoparticles. Singapore: Springer Nature Singapore; 2022. pp. 45-59. DOI: 10.1007/978-981-16-6819-7_3
- 29. Khoshnamvand M, Hao Z, Fadare OO, Hanachi P, Chen Y, Liu J. Toxicity of biosynthesized silver nanoparticles to aquatic organisms of different trophic levels. Chemosphere. 2020; 258 :127346. DOI: 10.1016/j.chemosphere.2020.127346
- 30. Rahimi HR, Doostmohammadi M. Nanoparticle synthesis, applications, and toxicity. In: Applications of Nanobiotechnology. London, UK: IntechOpen; 2020. DOI: 10.5772/intechopen.87973
- 31. Jamkhande PG, Ghule NW, Bamer AH, Kalaskar MG. Metal nanoparticles synthesis: An overview on methods of preparation, advantages and disadvantages, and applications. Journal of Drug Delivery Science and Technology. 2019; 53 :101174. DOI: 10.1016/j.jddst.2019.101174
- 32. Ying S, Guan Z, Ofoegbu PC, Clubb P, Rico C, He F, et al. Green synthesis of nanoparticles: Current developments and limitations. Environmental Technology and Innovation. 2022; 26 :102336. DOI: 10.1016/j.eti.2022.102336
- 33. Landge S, Ghosh D, Aiken K. Solvent-Free Synthesis of Nanoparticles, Green Chemistry. Amsterdam, Netherlands: Elsevier; 2018. pp. 609-646. DOI: 10.1016/B978-0-12-809270-5.00022-4
- 34. Kim D, Shin K, Kwon SG, Hyeon T. Synthesis and biomedical applications of multifunctional nanoparticles. Advanced Materials. 2018; 30 (49):1802309. DOI: 10.1002/adma.201802309
- 35. Halamoda-Kenzaoui B, Vandebriel RJ, Howarth A, Siccardi M, David CAW, Liptrott NJ, et al. Methodological needs in the quality and safety characterisation of nanotechnology-based health products: Priorities for method development and standardisation. Journal of Controlled Release. 2021; 336 :192-206. DOI: 10.1016/j.jconrel.2021.06.016
- 36. Anastas PT, Warner JC. Green Chemistry: Theory and Practice. Oxford [England], New York: Oxford University Press; 1998. p. 135
- 37. Singh J, Dutta T, Kim KH, Rawat M, Samddar P, Kumar P. ‘Green’ synthesis of metals and their oxide nanoparticles: Applications for environmental remediation. Journal of Nanbiotechnology. 2018; 16 (1):84. DOI: 10.1186/s12951-018-0408-4
- 38. Singh A, Gautam PK, Verma A, Singh V, Shivapriya PM, Shivalkar S, et al. Green synthesis of metallic nanoparticles as effective alternatives to treat antibiotics resistant bacterial infections: A review. Biotechnology Reports. 2020; 25 :e00427. DOI: 10.1016/j.btre.2020.e00427
- 39. Makarov VV, Love AJ, Sinitsyna OV, Makarova SS, Yaminsky IV, Taliansky ME, et al. “Green” nanotechnologies: Synthesis of metal nanoparticles using plants. Acta Naturae. 2014; 6 (1):35-44. DOI: 10.32607/20758251-2014-6-1-35-44
- 40. Hebbalalu D, Lalley J, Nadagouda MN, Varma RS. Greener techniques for the synthesis of silver nanoparticles using plant extracts, enzymes, bacteria, biodegradable polymers, and microwaves. ACS Sustainable Chemistry & Engineering. 2013; 1 (7):703-712. DOI: 10.1021/sc4000362
- 41. Agarwal H, Venkat Kumar S, Rajeshkumar S. A review on green synthesis of zinc oxide nanoparticles – An eco-friendly approach. Resource-Efficient Technologies. 2017; 3 (4):406-413. DOI: 10.1016/j.reffit.2017.03.002
- 42. Velusamy P, Kumar GV, Jeyanthi V, Das J, Pachaiappan R. Bio-inspired green nanoparticles: Synthesis, mechanism, and antibacterial application. Toxicological Research. 2016; 32 (2):95-102. DOI: 10.5487/TR.2016.32.2.095
- 43. Vetchinkina E, Loshchinina E, Kupryashina M, Burov A, Pylaev T, Nikitina V. Green synthesis of nanoparticles with extracellular and intracellular extracts of basidiomycetes. PeerJ. 2018; 6 :e5237. DOI: 10.7717/peerj.5237
- 44. Das VL, Thomas R, Varghese RT, Soniya EV, Mathew J, Radhakrishnan EK. Extracellular synthesis of silver nanoparticles by the Bacillus strain CS 11 isolated from industrialized area. 3 Biotechnology. 2014; 4 (2):121-126. DOI: 10.1007/s13205-013-0130-8
- 45. Singh P, Kim YJ, Zhang D, Yang DC. Biological synthesis of nanoparticles from plants and microorganisms. Trends in Biotechnology. 2016; 34 (7):588-599. DOI: 10.1016/j.tibtech.2016.02.006
- 46. Messaoudi O, Bendahou M. Biological synthesis of nanoparticles using endophytic microorganisms: Current development. In: Nanotechnology and the Environment. London, UK: IntechOpen; 2020. DOI: 10.5772/intechopen.93734
- 47. Mukherjee P, Ahmad A, Mandal D, Senapati S, Sainkar SR, Khan MI, et al. Fungus-mediated synthesis of silver nanoparticles and their immobilization in the mycelial matrix: A novel biological approach to nanoparticle synthesis. Nano Letters. 2001; 1 (10):515-519. DOI: 10.1021/nl0155274
- 48. Syed A, Ahmad A. Extracellular biosynthesis of platinum nanoparticles using the fungus fusarium oxysporum . Colloids and Surfaces. B, Biointerfaces. 2012; 97 :27-31. DOI: 10.1016/j.colsurfb.2012.03.026
- 49. Riddin TL, Gericke M, Whiteley CG. Analysis of the inter- and extracellular formation of platinum nanoparticles by fusarium oxysporum f. sp. lycopersici using response surface methodology. Nanotechnology. 2006; 17 (14):3482-3489. DOI: 10.1088/0957-4484/17/14/021
- 50. Rana A, Yadav K, Jagadevan S. A comprehensive review on green synthesis of nature-inspired metal nanoparticles: Mechanism, application and toxicity. Journal of Cleaner Production. 2020; 272 :122880. DOI: 10.1016/j.jclepro.2020.122880
- 51. Dahoumane SA, Yéprémian C, Djédiat C, Couté A, Fiévet F, Coradin T, et al. A global approach of the mechanism involved in the biosynthesis of gold colloids using micro-algae. Journal of Nanoparticle Research. 2014; 16 (10):2607. DOI: 10.1007/s11051-014-2607-8
- 52. Carrillo-López LM, Soto-Hernández RM, Zavaleta-Mancera HA, Vilchis-Néstor AR. Study of the performance of the organic extracts of Chenopodium ambrosioides for Ag nanoparticle synthesis. Journal of Nanomaterials. 2016; 2016 :1-13. DOI: 10.1155/2016/4714162
- 53. Dauthal P, Mukhopadhyay M. Noble metal nanoparticles: Plant-mediated synthesis, mechanistic aspects of synthesis, and applications. Industrial and Engineering Chemistry Research. 2016; 55 (36):9557-9577. DOI: 10.1021/acs.iecr.6b00861
- 54. Saim AK, Kumah FN, Oppong MN. Extracellular and intracellular synthesis of gold and silver nanoparticles by living plants: A review. Nanotechnology for Environmental Engineering. 2021; 6 (1):1. DOI: 10.1007/s41204-020-00095-9
- 55. Naikoo GA, Mustaqeem M, Hassan IU, Awan T, Arshad F, Salim H, et al. Bioinspired and green synthesis of nanoparticles from plant extracts with antiviral and antimicrobial properties: A critical review. Journal of Saudi Chemical Society. 2021; 25 (9):101304. DOI: 10.1016/j.jscs.2021.101304
- 56. Álvarez-Chimal R, García-Pérez VI, Álvarez-Pérez MA, Tavera-Hernández R, Reyes-Carmona L, Martínez-Hernández M, et al. Influence of the particle size on the antibacterial activity of green synthesized zinc oxide nanoparticles using Dysphania ambrosioides extract, supported by molecular docking analysis. Arabian Journal of Chemistry. 2022; 15 (6):103804. DOI: 10.1016/j.arabjc.2022.103804
- 57. Thanh NTK, Maclean N, Mahiddine S. Mechanisms of nucleation and growth of nanoparticles in solution. Chemical Reviews. 2014; 114 (15):7610-7630. DOI: 10.1021/cr400544s
- 58. Handayani W, Ningrum AS, Imawan C. The role of pH in synthesis silver nanoparticles using Pometia pinnata (Matoa) leaves extract as bioreductor. Journal of Physics: Conference Series. 2020; 1428 (1):012021. DOI: 10.1088/1742-6596/1428/1/012021
- 59. De Oliveira RC, Amoresi RAC, Marana NL, Zaghete MA, Ponce M, Chiquito AJ, et al. Influence of synthesis time on the morphology and properties of CeO 2 nanoparticles: An experimental–Theoretical study. Crystal Growth and Design. 2020; 20 (8):5031-5042. DOI: 10.1021/acs.cgd.0c00165
- 60. Kuppusamy P, Yusoff MM, Maniam GP, Govindan N. Biosynthesis of metallic nanoparticles using plant derivatives and their new avenues in pharmacological applications – An updated report. Saudi Pharmaceutical Journal. 2016; 24 (4):473-484. DOI: 10.1016/j.jsps.2014.11.013. DOI: 10.1016/j.molstruc.2016.12.069
- 61. Tippayawat P, Phromviyo N, Boueroy P, Chompoosor A. Green synthesis of silver nanoparticles in aloe vera plant extract prepared by a hydrothermal method and their synergistic antibacterial activity. PeerJ. 2016; 4 . DOI: 10.7717/peerj.2589
- 62. Amina M, Al Musayeib NM, Alarfaj NA, El-Tohamy MF, Oraby HF, Al Hamoud GA, et al. Biogenic green synthesis of MgO nanoparticles using Saussurea costus biomasses for a comprehensive detection of their antimicrobial, cytotoxicity against MCF-7 breast cancer cells and photocatalysis potentials. PLoS One. 2020; 15 (8). DOI: 10.1371/journal.pone.0237567
- 63. Ghorbani HR. Extracellular synthesis of copper nanoparticles using culture supernatants of salmonella typhimurium . Oriental Journal of Chemistry. 2015; 31 (1):527-529. DOI: 10.13005/ojc/310165
- 64. Singh AK, Tiwari R, Kumar V, Singh P, Riyazat Khadim SK, Tiwari A, et al. Photo-induced biosynthesis of silver nanoparticles from aqueous extract of Dunaliella salina and their anticancer potential. Journal of Photochemistry and Photobiology, B: Biology. 2017; 166 :202-211. DOI: 10.1016/j.jphotobiol.2016.11.020
- 65. Nava OJ, Luque PA, Gómez-Gutiérrez CM, Vilchis-Nestor AR, Castro-Beltrán A, Mota-González ML, et al. Influence of Camellia sinensis extract on zinc oxide nanoparticle green synthesis. Journal of Molecular Structure. 2017; 1134 :121-125
- 66. Duan H, Wang D, Li Y. Green chemistry for nanoparticle synthesis. Chemical Society Reviews. 2015; 44 (16):5778-5792. DOI: 10.1039/C4CS00363B
- 67. Razavi M, Salahinejad E, Fahmy M, Yazdimamaghani M, Vashaee D, Tayebi L. Green Chemical and Biological Synthesis of Nanoparticles and their Biomedical Applications. Green Processes for Nanotechnology. Cham: Springer International Publishing; 2015. pp. 207-235. DOI: 10.1007/978-3-319-15461-9_7
- 68. Badria F, AbouHabieb M, Bar FA. Synthesis of Nanoparticles Using Green Chemistry Green Synthesis. 1. Auflage ed. München: Grin Verlag; 2019
- 69. Chopra H, Bibi S, Singh I, Hasan MM, Khan MS, Yousafi Q , et al. Green metallic nanoparticles: Biosynthesis to applications. Frontiers in Bioengineering and Biotechnology. 2022; 10 :874742. DOI: 10.3389/fbioe.2022.874742
- 70. Michael A, Singh A, Roy A, Islam MR. Fungal- and algal-derived synthesis of various nanoparticles and their applications. Bioinorganic Chemistry and Applications. 2022; 2022 :1-14. DOI: 10.1155/2022/3142674
- 71. Raj S, Trivedi R, Soni V. Biogenic synthesis of silver nanoparticles, characterization and their applications—A review. Surfaces. 2021; 5 (1):67-90. DOI: 10.3390/surfaces5010003
- 72. Kebede MA, Wubieneh TA, Yohannes YB, Shah KJ. Green synthesis of zinc oxide from aqueous fruit extract of Dovyalis abyssinica (Koshem) and application for water purification. Ethiopian Journal of Science and Technology. 2023; 16 (1):1-12. DOI: 10.4314/ejst.v16i1.1
© The Author(s). Licensee IntechOpen. This chapter is distributed under the terms of the Creative Commons Attribution 3.0 License , which permits unrestricted use, distribution, and reproduction in any medium, provided the original work is properly cited.
Continue reading from the same book
Advances in green chemistry.
Edited by Kinjal Shah
Published: 31 January 2024
By Faisal Nawaz, Irum Jamil and Bushra Shakoor
By Anja Verbič, Gregor Primc, Martin Šala and Marija ...
41 downloads
166 downloads

An official website of the United States government
The .gov means it’s official. Federal government websites often end in .gov or .mil. Before sharing sensitive information, make sure you’re on a federal government site.
The site is secure. The https:// ensures that you are connecting to the official website and that any information you provide is encrypted and transmitted securely.
- Publications
- Account settings
Preview improvements coming to the PMC website in October 2024. Learn More or Try it out now .
- Advanced Search
- Journal List
- PMC10254633

Biosynthesis of Nanoparticles from Various Biological Sources and Its Biomedical Applications
Gopalu karunakaran.
1 Institute for Applied Chemistry, Department of Fine Chemistry, Seoul National University of Science and Technology, 232 Gongneung-ro, Nowon-gu, Seoul 01811, Republic of Korea
Kattakgoundar Govindaraj Sudha
2 Department of Biotechnology, K. S. Rangasamy College of Arts and Science (Autonomous), Tiruchengode 637215, Tamil Nadu, India
3 Department of Periodontics, Saveetha Dental College and Hospital, Saveetha Institute of Medical and Technical Sciences (SIMATS), Chennai 600077, Tamil Nadu, India
Eun-Bum Cho
Associated data.
Not applicable.
In the last few decades, the broad scope of nanomedicine has played an important role in the global healthcare industry. Biological acquisition methods to obtain nanoparticles (NPs) offer a low-cost, non-toxic, and environmentally friendly approach. This review shows recent data about several methods for procuring nanoparticles and an exhaustive elucidation of biological agents such as plants, algae, bacteria, fungi, actinomycete, and yeast. When compared to the physical, chemical, and biological approaches for obtaining nanoparticles, the biological approach has significant advantages such as non-toxicity and environmental friendliness, which support their significant use in therapeutic applications. The bio-mediated, procured nanoparticles not only help researchers but also manipulate particles to provide health and safety. In addition, we examined the significant biomedical applications of nanoparticles, such as antibacterial, antifungal, antiviral, anti-inflammatory, antidiabetic, antioxidant, and other medical applications. This review highlights the findings of current research on the bio-mediated acquisition of novel NPs and scrutinizes the various methods proposed to describe them. The bio-mediated synthesis of NPs from plant extracts has several advantages, including bioavailability, environmental friendliness, and low cost. Researchers have sequenced the analysis of the biochemical mechanisms and enzyme reactions of bio-mediated acquisition as well as the determination of the bioactive compounds mediated by nanoparticle acquisition. This review is primarily concerned with collating research from researchers from a variety of disciplines that frequently provides new clarifications to serious problems.
1. Introduction
1.1. history.
The word “nano” was derived from the Greek word “nanos,” it means “little,” and it is the cognomen of the one-thousandth bit (109). Nanoparticles (NPs) confer a solid colloidal particle with at least one dimension ranging from 1 to 100 nm [ 1 ]. However, the majority of materials that are applied in drug delivery are in the range of 100–200 nm. Because of their unique electronic structure, massive conductivity, wide exterior position, and quantum size significance, these particles can change their chemical and physical properties. Nanoparticles are used in a variety of applications today, including anti-viral, cosmetics, electronics, and textiles [ 2 , 3 ]. Researchers have discovered numerous beneficial effects of nanotechnology, and it now plays an important role globally. Nanotechnology has created several material industries, including the food industry, and now its role in the biomedical field has increased, providing better results in the future medicine field [ 4 , 5 ].
1.2. Different Properties of Nanoparticles
The nanoparticles’ small size has several extraordinary properties that play an important role in various reactions [ 6 ]. Nanoparticles are nanoscale systems that are gaining popularity among researchers due to their ability to develop a wide range of shapes and sizes that can be used in a variety of advanced biotechnological applications. Nanoparticles have distinct physicochemical and optoelectronic properties that may be unique properties of implementations such as electronics, pharmaceutical compounds, chemical sensors, catalysts, antimicrobial agents, clinical diagnostics, and mapping. These features provide nanoparticles with more specific and significant effects than bulk particles or unique individual molecules. Due to these properties, improved electrical transport, stimulated roughness, and stability make it most effective for the medicine, textile, defense, agriculture, food, cosmetics, and space sectors [ 7 ]. Nanoparticles exhibit tremendous shape and size characteristics, as well as a broad spectrum of applications when compared to other bulk materials, and are used for broad-scope implementations [ 8 ].
1.3. Several Approaches for Nanoparticle Synthesis
The top-down method, in which the synthesis begins with breaking bulk material into pieces and the development of nanoparticles, is the most widely used approach for nanoparticle synthesis. The bottom-up method involves gathering atoms and molecules to develop various nanoparticles. The most effective merit of the top-down method is that it facilitates the development of nanoparticles in large numbers in a short amount of time. However, the main merit of the bottom-up method is that it leads to the formation of nanoparticles with defined crystallographic properties and a higher specific surface area ( Figure 1 ) [ 9 ].

Schematic representation of top-down and bottom-up method for the obtaining of nanoparticles.
A top-down approach for synthesizing the defined shape is not feasible. However, by using a bottom-up approach, waste components can be eliminated and fewer nanoparticles can be created. The chemical reduction method is one of the main approaches for obtaining nanoparticles using the bottom-up method. Commonly, the nanoparticle synthesis method might be categorized into three parts: chemical, physical, and bio-mediated approaches [ 10 ]. Additionally, this method was suggested as the most significant and widely used approach for synthesizing nanoparticles.
Temperature, pressure, and energy are all used in the physical approach to obtain NPs [ 11 ]. By the chemical method, NPs are obtained through sol–gel, atomic condensation, chemical etching, laser pyrolysis, spray-mediated pyrolysis, and sputtering. The morphologies of the nanoparticles can be altered by chemical and reaction ratios. After the synthesis, the obtained nanoparticles might encounter difficulties in terms of bioaccumulation, the toxic nature, regrowth, reuse, and recycling [ 12 , 13 ]. However, green-approach-mediated synthesized NPs have proven to be non-toxic [ 14 ].
The biosynthesis of nanoparticles with distinct sizes and shapes has been used for a variety of applications in biomaterials science [ 15 , 16 ]. Nanoparticles have been developed for several applications in the pharmaceutical field to treat several viral and bacterial diseases [ 4 ]. The biosynthesis approach has various merits compared to other classical synthesis protocols because of the capacity for bulk production under eco-friendly approaches. The vast biological diversity and easy sources of plant output have been thoroughly investigated for the amalgamation of nanomaterials. These biologically synthesized nanomaterials have significant applications in various fields such as diagnosis, treatment, manipulation of surgical nanodevices, and other product formations. Nanomedicine has shown promising clinical results in the management of diverse chronic diseases. Moreover, eco-friendly methods of obtaining NPs were marked as fundamental materials for the upcoming generations to protect from several diseases [ 1 ].
1.4. Antibacterial and Antiviral Properties, the Importance of Zeta Potential, and Mode of Action of Nanoparticles
Greenly synthesized metal and metal oxide NPs such as Au, Ag, Pt, ZnO, and Se NPs are used in pharmaceutical products, cosmetics, antimicrobial applications, and medical applications. Bio-manipulated nanoparticles are currently being used in clinical settings for the diagnosis, treatment, transportation, and manipulation of specific medicines [ 3 ]. Metallic NPs such as Ag, Au, Zn, Pt, Fe, Ni, Cu, Mg, Ti, and their oxides have provided clear ideas of the process of obtaining them for various decades [ 4 ]. The variously obtained nanoparticles show several uses in different fields, such as chemical sensing, optical elements, catalysis, pharmaceutical agents, and antibacterial agents.
Silver nanoparticles (AgNPs) are highly reactive and are coupled with tissue proteins, causing morphology changes in the bacterial nuclear and cell membranes, resulting in cell breakage and mortality [ 17 ]. The destruction of the cell wall takes place when the nanoparticles come into contact with the cell wall. Maillard et al., gave a detailed explanation of the role of the zeta potential in antibacterial activity [ 18 ]. According to this review, the zeta potential is a very useful tool to understand the viability and membrane permeability of bacteria and how the zeta potential of materials is important to develop new advanced antibacterial materials. In a study, ZnO nanoparticles were used to evaluate their antimicrobial properties using different zeta potential bacteria from −14.7 to −23.6 mV. In this study, it was shown that Gram-positive bacteria have a lower zeta potential value, whereas Gram-negative bacteria have a higher zeta potential value [ 19 ]. In this study, the ZnO zeta potential value was altered to see the effect on different bacteria. An attempt was made to neutralize the opposite zeta potential, which is the positive and negative value, and to thus have a higher positive charge. ZnO nanoparticles had a higher antibacterial activity against negatively charged Gram-negative bacteria. Thus, this study confirms that the zeta potential plays an important role in the design of antibacterial agents. Ag can also interact with bacterial RNA and DNA by damaging them and suppressing bacterial growth. Ag has stronger antifungal and antiviral properties. Ag metal and Ag coatings are applied in considerable amounts and have no side-effects in the human system against various diseases, due to viruses, bacteria, fungi, and yeast [ 3 ].
AgNPs exhibit better efficiency toward bacteria and less toxicity to the human system. Commonly, Ag ions might be attached to negative-power molecules such as DNA, RNA, and proteins. Silver NPs, obtained through biological methods, exhibit anti-bacterial activity toward bacteria, including the methicillin-resistant Staphylococcus aureus ( S. aureus ) [ 4 ].
Yazdanian and coauthors showed that AuNPs were also found to be an excellent antibacterial obtained from Alternanthera philoxeroides -mediated green synthesis. The obtained nanoparticles were around 72 nm in size [ 3 ]. CuNP was synthesized using Cardiospermum halicacabum as a biosynthesis, and the synthesized nanoparticles were 30–40 nm in size with a hexagonal shape. The obtained CuNP was found to inhibit the bacterial cell wall and inhibit the growth of bacteria [ 3 ]. FeNPs were also obtained using Euphorbia hirta through green synthesis, and the obtained nanoparticles were 25–80 nm in size with a cavity-like structure. They showed excellent antibacterial and antifungal activities against various pathogens [ 3 ]. TiNP obtained using Azadirachta indica was found to be around 18 nm with crystalline structures and to possess outstanding antibacterial activity against various bacteria [ 3 ]. Further, ZnONP obtained using Costus pictus was found to be around 40 nm in size and have excellent antibacterial and antifungal activities [ 3 ].
Viruses are a major threat to humans, as about 60% of major illnesses are due to virus-based infections [ 20 ]. The virus infects through respiratory or enteric channels. Viruses are transferred in different ways, such as by surface contact, direct transfer from an infected person to another person through secretions, aerosol particulates, and inanimate surfaces [ 21 , 22 , 23 , 24 ]. There is a demand for the development of antiviral agents to control infections caused by viruses [ 21 , 22 , 23 , 24 ]. According to Choudhary et al. (2020), tiny AgNPs are acceptable to human immune viruses, and the coupling of Ag nanoparticles of 5 nm with the gp120 protein of the HIV suppresses the virus by targeting itself in the host tissue [ 22 ]. Gold nanoparticles of around 17 nm were also inhibiting HIV-1 infections through fusion [ 21 ].
Copper iodide nanoparticles of around 160 nm were found to inhibit the Feline calicivirus infection by ROS generation and also by the oxidation of capsid proteins [ 21 ]. Cuprous oxide nanoparticles of around 45 nm were found to inhibit the Hepatitis C virus infection by preventing the HCV virus’s entry through attachment mechanisms [ 21 ]. Iron oxide nanoparticles of 10–15 nm were also found to be very effective in inhibiting the H1N1 influenza virus infection by inactivating the cellular proteins by interacting with the proposed -SH functional group [ 21 ]. ZnO nanoparticles of 16 to 20 nm were also found to be effective against H1N1 influenza, which inhibits the proliferation of the virus [ 21 ]. Up until now, various metal nanoparticles have been obtained and analyzed for their different biomedical applications. Hence, in this review, we describe the various synthesis methods for NPs and their biological mechanisms to feature their various biomedical applications.
2. Different Types of Nanoparticles
Generally, nanoparticles are categorized into three kinds: organic (polymers, proteins, and lipids), inorganic (salts and metals), and hybrid (nanofoams) ( Figure 2 ). Organic nanoparticles or polymers were identified as dendrimers, micelles, liposomes, and ferritin. These particles are not toxic or biocatalytic, and a few particles such as micelles and liposomes include a hollow core, which is marked as a nanocapsule, and are sensitive to electromagnetic rays such as light and heat [ 25 ]. Numerous organic chemicals are used in different pharmaceutical products, such as dyes, flavors, inks, and household products. In several of these products, the organic chemicals are diluted for development or chemically altered to enhance the particle properties. Chemicals are not dispersed in a liquid that is essential for development; their properties and applications are effectively restricted [ 26 ].

Schematic representation of various types of nanoparticles.
The pharmaceutical outcome has limitations in bioavailability and efficiency because it is not soluble in water. This might be due to limitations in the formation of new drugs or the area of recent medicine [ 27 ]. The same can be said for nutraceuticals, biocides, and a variety of other important essential compounds. For developing a tiny dilution of organic components, non-soluble particles might be used to act as purely dispersed molecules. It is not essential to develop new chemicals or utilize burnable, toxic, or vaporous solutions. This selection was necessary for the development and formation of new results due to the extent of chemical components that must be accessible, which gives high importance to a novel and merciless outline. Organic nanoparticles may provide a solid material coupled with organic components such as polymers or lipids, particularly at scales ranging from 10 nm to 1 m. The particles attained more intention as compared to inorganic particles, where numerous studies and trade speculations were being formed [ 28 ].
In recent years, the pharmaceutical industry has conducted interesting research on organic nanoparticles, as a result of which nanomedicine has enabled the formation of new particles and the purification of better-developed methods. The formation of dendrimers, protein connections, DNA-transferring devices, liposomes, and shell-over-coupled brick co-polymer micelles may be noted for nanoparticles synthesized from the “bottom-up” method of transferring active molecules. A “top-down” approach has gained more researcher interest, as have methods such as wet nano-milling to grind large materials and attain material separation with sub-micron maximum material cadence. Various wet methods to develop colloidal dilutions form liquid mixtures, which critically depend on the particular excretion of the oil phase of an amalgam and ensure solidification through precipitation, enclosure, and crystallization of any organic particles that are dispersed within the solvents dropwise [ 29 ].
All of these techniques have been proven to be purely generic, and they may have been limited to specific classes of particles classified by their chemical reactivity or physical characteristics. Organic suspension environments, which might be diluted by dropwise differentiation from their inorganic constituents, do not hold organic nanoparticles tenaciously in the environment for a lengthy manufacturing process [ 30 ].
Organic and inorganic hybrid nanoparticles are most effective at attracting people’s attention. Combining inorganic and organic substances in the nanoscopic range is not a novel idea. Nature has a plethora of hybrid particles such as nacre, bone, and corals that interact with biogenic substances and inorganic agents to transfer hybrid biomaterials for the benefit of humans [ 31 ]. Inorganic and organic combined nanomaterials are used in various fields, including optics, optoelectronics, coating, and bio-clinical applications. In common terms, polymer substances have critical structural properties that can be manipulated for their mechanical properties and accessibility at the end products, whereas the inorganic substances might induce unique properties such as luminescence, magnetism, and catalytic features to induce the heating and mechanical features in the organic materials [ 32 ].
Metal and metal-based nanoparticles are frequently classified as inorganic nanomaterials. Nanoparticles have various advantages, such as their small size and higher surface area, which have stimulated the interest of researchers in recent years, and they are now being used in a variety of biological and engineering fields [ 33 ]. In comparison to organic and hybrid nanoparticles, inorganic nanoparticles are now modified with several chemical features that allow them to bind with antibodies, drugs, and ligands, launching a different range of significant implementations in filtration, drug delivery, biotechnology, and as carriers for gene-mediated transfer with improved disease mapping [ 34 ]. However, this mapping has developed in recent years as magnetic mapping, computed tomography, ultrasound, and Raman spectroscopy have been used to map several disease stages. Nanoparticles such as gold, titanium, and silver are developed for use in numerous mapping applications. Furthermore, nanoshells and nanocapsules have been created to use mapping methods in a broad implementation. In the past few decades, nanoparticles such as Au, Ag, and magnetic nanoparticles have been effectively utilized for their use in disease treatment and as diagnostic agents. The future usage of inorganic nanoparticles such as silicas, quantum dots, titania, and many catalysts is not an inquiry but will give nano-solutions a more fanciful time in the numerous non-dispersible or imperfectly soluble organic components that are applied over various improved technologies and materials outlined [ 35 ].
Various types of inorganic nanomaterials and various methods of obtaining them have made it possible to manipulate new drug delivery systems. Several significant issues should be considered before converting these inorganic nanomaterials into medicinal products. Differentiated from the better-organized organic nanoparticles, the medicinal conversion of inorganic nanomaterials for drug delivery is still under stable argument because of the absence of a stronger proof and data relating to biological safety, especially the bio-catalytic features, elimination methods, and long-use toxicity assays to support its in vitro and in vivo biological safety. The biocompatible inorganic particle-dependent nanomaterials provide an unrivaled opportunity and demonstrate a better scope for effective clinical implementation in a variety of diseases [ 36 ].
3. Various Biosources Are Used to Synthesize Nanoparticles
3.1. green synthesis of nanoparticles.
Green-mediated nanoparticle synthesis is a low-cost, environmentally friendly method with no toxic properties. This method uses various stabilizing and reducing substances, such as plants, microbes, and some natural agents, to develop NPs. The green-mediated obtaining of nanoparticles has gained popularity due to their low cost, non-toxicity, and high stability. The green-mediated synthesizing approach was an eco-friendly method to manipulate nanoparticles that did not cause toxic effects on the environment or human health. The conventional approach might manipulate nanoparticles in large amounts with defined shapes and sizes. Furthermore, these approaches necessitate massive economies, are difficult, and adhere to outdated protocols. The green synthesis method has numerous advantages over chemical and physical methods, including ease of development, simplicity, low cost, and low waste in the development of NPs [ 37 , 38 ].
The green-mediated approach to obtaining NPs is differentiated, but organisms or their extracts are easily responsive to metallic salt, and biological stabilization was analyzed to turn metal into NPs. The formation of nanoparticles by organisms was a green method that used fungi, bacteria, and viruses’ enzymes and secondary metabolites. These kinds of organisms provide primary substances for the synthesis and manipulation of better-organized nanoparticles [ 39 ]. For differentiation, the microbially mediated obtaining method was a significant one, and plants might be applied as a beneficial method for nanoparticle formation. For obtaining nanoparticles, it might be easier to use plant extracts. Furthermore, plant extracts may reduce metallic ions, allowing microbes to easily form and stabilize metallic nanoparticles [ 40 , 41 ]. Plant extracts and various components such as proteins, polysaccharides, amino acids, and phytochemicals such as flavonoids, alkaloids, tannins, and polyphenols are appearing that might stabilize and reduce the nanoparticles ( Figure 3 ).

Pictorial presentation of biologically mediated synthesis of nanoparticles.
3.2. Plant-Based Synthesis of Nanoparticles
Plant parts such as leaves, stems, flowers, bark, roots, fruits, vegetables, and shoots are used as a primary compound for the synthesis process of nanoparticles. A variety of plant leaf extracts are used for synthesis, which includes bioactive components that occur during the synthesis process using an eco-friendly approach [ 42 , 43 ]. Plant extracts are used to obtain metallic nanoparticles, but recent research has demonstrated an effective and unique method for obtaining nanoparticles via well-managed purified plant components [ 44 , 45 ]. Plant bioactive components are used as effective cancer drugs to treat a variety of cancers and dangerous diseases ( Table 1 ).
Biosynthesis of nanoparticles using plant extract.
As a result, the downstream processing-related scale-up approach effectively stimulated and reduced manipulation duration. Many studies have suggested the use of extracted phenolic components such as secondary metabolites, sugars, and proteins for the synthesis of various metallic nanoparticles. Plants or their extracts, particularly flavonoids, have been used for the bioremediation of metals for a lengthy period. Plant metabolite flavonoids, such as quercetin, were applied to biosynthesize copper and silver nanoparticles in micelle suspension, which have better antibacterial activity and a higher surface area. The Desmodium triflorum plants were given silver ions in an agar suspension, followed by shooting, resulting in an increase in the concentration of silver ions in the tissue, and this clearly shows the uptake of silver ions by plants [ 46 ]. The detoxification, capping of silver, and development of AgNPs in the tissue were observed. The different nanoparticles developed with Chrysophyllum oliviforme plants are in the range from 20 to 50 nm in diameter and have less polydispersity, which reveals the effective in vivo synthesis of nanoparticles. These materials allow for the development of a higher positioning of materials, with the possibility of synthesis [ 47 ]. The extract of Veronica amygdalina was observed for the bioconversion of silver ions. Faster development of silver nanoparticles with a merit size of 15 nm was achieved, as was a controlled scale of material size between 2 and 18 nm. Terpenoids such as geraniol, linalool, and citronellal found in geranium leaf extracts are feasible reducing substances for silver ions [ 48 ].
3.3. Amalgamation of Nanoparticles Using Marine Algae
For the amalgamation of marine algae nanoparticles, different literature data are available ( Table 2 ). The brown seaweed Sargassum wightii was stated to have the ability to synthesize nanoparticles with a defined range between 8 and 12 nm [ 62 ]. The synthesis of nanoparticles using seaweed ( Fucus vesiculosus ) is reported to have the capacity for Au bio-absorption and bio-reduction reactions, which are useful to extract Au from dispersing hydrometallurgical solutions and electronic scrapes for obtaining NPs with different shapes and sizes ( Figure 3 ). The synthesized nanoparticles made from algae extracts produce that stabilizing effect on cotton fabrics with excellent antibacterial activity. G. acerosa was stated to include the significance of obtaining antifungal Ag NPs [ 62 , 63 ].
Biosynthesis of nanoparticles using marine algae.
3.4. Bacteria-Mediated Synthesis of Nanoparticles
Multicellular and single-cellular organisms are used to produce materials that might be intracellular or extracellular. The bacterial enzymes occur through an intracellular signaling mechanism for the conversion of ions into NPs [ 67 ]. The main merits of the bacterially mediated amalgamation of nanoparticles are that it is easy to use and better controls the whole process of biosynthesis. Following genetic engineering methods, the development could be formed quickly for effective purposes such as reducing toxic effects and achieving defined nanoparticle synthesis [ 68 ]. This method also has some restrictions, such as downstream reactions, a cost-effective but laborious approach, and the need to maintain control over size and shape.
Moreover, metal nanoparticles are synthesized through intra- and extracellular bacterial extracts. The extraction process of nanoparticles uses mainly cellular extract via the downstream method. In this protocol, a diverse group of sulfate-reducing bacteria, such as Acinetobacter calcoaceticus and Desulfovibrio desulfuricans, is used for the controlled development of nanoparticles of controlled size ( Figure 3 and Table 3 ). These bacteria have a unique feature that gives them the capability to convert precursor solutions into nanoparticles. At 37 °C and a neutral pH, the reducing bacterium Rhodopseudomonas capsulate was used to obtain nanoparticles by using elemental nanoparticles [ 69 ].
Biosynthesis of nanoparticles using bacteria.
3.5. Fungi-Mediated Synthesis of Nanoparticles
Various fungal species are used to obtain different NPs ( Table 4 ). The obtained nanoparticles, which include various microorganisms and fungi, are more effective prokaryotes. Fungi have some advantages over other microbial methods of obtaining nanoparticles in that it is easy to develop media, scale-up formation is easier, downstream reactions are easier, an increased number of proteins are generated, and biomass is simple to prepare. However, fungal enzymes increase the number of nanoparticles synthesized and have achieved the reductive properties required for the production of stable nanoparticles. Commonly, extracellularly produced nanoparticles are low in toxicity. Nanoparticles are developed extracellularly at 37 °C with a wide range from 15 to 30 nm via Fusarium oxysporum [ 79 ]. Moreover, this research provides that the obtaining of nanoparticles by fungal extracts would be through the processes of reduction and stabilization of substances [ 80 ].
Biosynthesis of nanoparticles using fungi.
The synthesis of fungal-associated nanoparticles is a hot topic in the scientific community. Fungi have been commonly used for the biosynthesis of nanoparticles and have attracted researchers’ attention for their ability to develop well-managed shapes and sizes of nanoparticles [ 88 ]. Fungi have been commonly used for the bio-mediated obtaining of nanoparticles, and better-managed dimensions might be obtained. Fungi, as they differentiate from bacteria, produce a higher ratio of NPs. Fungi excrete increased protein ratios, which effectively alter the formation of nanoparticles [ 89 ].
3.6. Actinomycetes-Mediated Synthesis of Nanoparticles
Actinomycetes have significant features similar to fungi and prokaryotes such as bacteria. It was concluded that the Ag and Au NPs are stabilized by proteins. Free amino acids or cysteine remain on proteins that have been coupled with Au NPs. Initially, gel electrophoresis reveals that the actinomycetes excrete four particular proteins of molecular aggregate in the range from 10 to 80 kDa. Hence, it was confirmed that the reduction and stabilization of the gold nanoparticles were brought about by various proteins present in them [ 90 ].
Thus, by developing various proteins and their ability to connect with various crystallographic features of gold nanocrystals, it is possible to develop complex shapes with manageable sizes. Actinomycetes have unique properties for which secondary metabolites can be used to create antibiotics. It was noted that actinomycetes play a significant role in the synthesis of metal nanoparticles [ 91 ]. An alkalothermophilic actinomycete obtained Au ions of 8 nm in size under extremely alkaline conditions and at constant temperatures. Rhodococcus sp. and Thermomonospora sp., both alkalotolerant actinomycetes, are used to produce gold nanoparticles. Intracellularly synthesized gold nanoparticles with diameters of 5 and 15 nm were also created. According to the findings, the ratio of NPs on the cell membrane was higher than on the cell barrier. The enzyme formed on the cell barrier and cell membrane was responsible for the formation of Au NPs. The cells were followed to develop nanoparticles, which revealed that the obtained nanoparticles were non-toxic to the cells [ 91 ].
3.7. Yeast-Mediated Synthesis of Nanoparticles
Biological reactions can manage the shape of materials better. In the log phase, Schizosaccharomyces pombe synthesized semiconductor nanocrystals. Extracellularly synthesized particles from microorganisms have a broad spectrum of benefits because protein-mediated interactions with the organisms make it easier for downstream reactions ( Figure 3 and Table 5 ). Yeasts, a group of ascomycetes in fungi, have been shown to have more significant capabilities for obtaining NPs. Au NPs have been obtained intracellularly through the fungus Verticillium luteoalbum. The ratio of material development and the scale range of the NPs could be areas to explore by controlling physical parameters such as pH and temperature.
The tremendous amount of published data showed that the entire yeast family might aggregate various heavy metals. They can aggregate potential ratios of increased toxic metals. Several reports concluded that extracellular polysaccharides or peptides, which manage the cell barrier of heavy metals against or to active efflux from the cell, are the various mechanisms formed through these species that overwhelm the toxic properties of heavy metals [ 92 ]. Direct administration of intracellular metal ions required yeast cells to eliminate negative or fetal outcomes. Toxicity to cells is reduced by maximizing metal ion retention or by exposing cells to metals that do not have the same potential properties as lead, mercury, and cadmium.
Yeast-mediated synthesis of nanoparticles.
The greater difference in size, particle arrangement, mono-dilution, and features is due to various mechanisms studied using yeast strains from different families for nanoparticle formation. GSH (glutathione) and two classes of metal-accepting coupling metallothioneins and phytochelatins (PC) were used to create a detoxification mechanism in yeast cells. In the majority of the yeast species reported, these molecules analyzed the pathway for the development of NPs and stabilized the compounds. Resistance was defined as the ability of yeast cells to change the absorption of metal ions into non-toxic compound polymer complexes. The yeast is commonly marked as “semi-transfer crystals” or “quantum semi-transfer crystals.” Current research has revealed that yeasts can also form other nanoparticles. In eukaryotes, yeast species are the most studied for bioprocesses [ 92 ].
Extracellular methods were used to obtain AgNPs from Cladosporium cladosporioides. It was stated that the proteins, organic acids, and polysaccharides excreted through fungus were capable of comparing various shapes and were used to regulate the formation of spherical crystals. Penicillium fellutanum was collected from coastal mangrove sediment, and using this sediment, AgNPs were synthesized by the extracellular synthesis method [ 92 ].
4. Biomedical Applications of Nanoparticles
4.1. antibacterial activity.
In disease-causing organisms, nanoparticles are designed to break the polymer sub-group of the cell membrane. The opposite role of NPs effectively disrupts protein formation and damages cell membranes in bacterial cells. Silver nanoparticles at higher concentrations induced membrane rupture compared with low concentrations and effectively damaged the bacterial cell wall ( Figure 4 ). R. apiculate -mediated silver nanoparticles showed a lower rate of growth than silver-nitrate-exposed bacterial cells, which could be because of particle size and increased exterior interaction, which resulted in induced cell membrane rupture and cell interruption [ 96 ].

Application of biologically mediated synthesized nanoparticles in biomedical field.
The current study reported on the significance of Pt nanoparticles in human health and their application to various disease targets via microbes. Microbes show effective resistance to many antibiotics [ 97 ]. However, the formation of nanoparticles along with strong antimicrobial activity was effective in the biomedical area. Several studies on metallic nanoparticles such as Ag, Au, Pt, Pd, ZnO, and TiO 2 have depicted a strong role in their antibacterial properties toward pathogenic microbes. Several metallic NPs, including Au, Ag, Pt, and ZnO, have strong cell death mechanisms. Another major feature of nanoparticles is their effective negative zeta potential, which induces antibacterial activity [ 98 ].
Apigenin from chamomile extract was used to create Pt nanoparticles with effective antibacterial potential against S. aureus. Previously, researchers discovered that the development of E. coli is suppressed by Pt electrolysis [ 99 ]. Another study found that a combination of Pt nanoparticles and partial ammonium had antibacterial effects by significantly suppressing Streptococcus mutans. Pt mixtures of polyamide S, including sulfones, reveal stronger antibacterial properties against E. coli and S. aureus [ 100 ].
Silver-Pt nanoparticles doped with particles of a size scale between 2 and 3 nm have induced significant antibacterial properties against K. pneumonia , P. aeruginosa , E. coli, and S. choleraesuis . Recent research has stated that the suppression of bacterial development is due to ATP generation and mitochondrial membrane stability. In addition, other reports stated that the implementation of polyvinylpyrrolidone-doped Pt nanoparticles with unique shapes and size scales ranging from 2 to 20 nm toward P. aeruginosa analyzed their antibacterial properties [ 101 ]. PVP-coupled Pt nanoparticles with diameters of 5.7 and 5.8 nm were exposed to S. aureus and E. coli Gram-negative bacteria, which are nanoparticles with small sizes that effectively suppress E. coli growth. Pt and Ag mixed nanoparticles are used for the reduction of graphene oxide (rGO) nanosheets, along with holes that lead to the induction of antibacterial properties against E. coli in the membrane between metal components, the rGO matrix, and bacteria. When combined with Pt NPs as a nano-mixture, polyvinylpyrrolidone (PVP) exhibits effective antibacterial properties against K. pneumonia , Lactococcus lactis, and E. coli [ 102 ]. Krishnaraj et al. (2010) stated that the A. indica -mediated Ag NPs strongly deplete the water-related pathogenic bacteria at a low concentration [ 103 ].
Based on the epidemic of infectious diseases caused by various pathogenic bacteria, there is an emerging need to discover novel antibacterial materials [ 104 , 105 ]. Several classes of NPs, such as Mg, Ti, Cu, or alginate, have effective antibacterial properties; gold and silver NPs have demonstrated a strong antiviral, antibacterial, and antifungal effect. The broad range of antibacterial properties of metallic nanoparticles, primarily gold and silver, encourages their use as disinfectants in the sterilization process of common water, medicine, food preparation, makeup, and various domestic items [ 106 ].
The biosynthesis of nanoparticles with antibacterial properties from fungi, bacteria, and algae to plant and tree root, leaf, bark, and tuber extract, and the mycosynthesis of Ag nanoparticles have excellent antibacterial activity against a variety of human-infection-causing organisms, including multidrug-resistant S. aureus and S. epidermidis. Similarly, the fungal strain Aspergillus was implanted for the extracellular synthesis of strong silver NPs with antibacterial properties against methicillin-resistant S. aureus and S. epidermidis [ 107 ]. Silver nanoparticles bio-mediated by Aspergillus oryzae filamentous mold showed antibacterial properties against S. aureus KCCM 12256. Bipolaris nodulosa fungal species might act as stabilizing substances for silver nitrate, which leads to the formation of silver nanoparticles in P. vulgaris and B. subtilis pathogens [ 108 ]. Silver nanoparticles were bio-mediated and obtained via gilled mushrooms of the species Pleurotus sajor-caju, which were effective against S. aureus [ 109 ].
Phoma glomerata fungal plant pathogens were studied for the production of silver nanoparticles and better antibacterial potential against S. aureus. Trichoderma viride -mold-species-bio-mediated vancomycin binds to nanoparticles and has demonstrated properties against vancomycin-resistant E. coli. Metal-reducing S. oneidensis was implemented for the biofabrication of Ag nanocrystals. Bacterial toxicity assays revealed that the obtained biogenic silver NPs had better bacterial properties toward S. oneidensis than other methods of obtaining colloidal silver NPs [ 110 ].
Several marine algae species are used to improve the antimicrobial properties of bio-mediated silver nanoparticles against human infectious pathogens P. vulgaricus , Klebsiella sp., E. coli , and P. aeruginosa . Garcinia mangostana leaf extract was tested in silver nanoparticles for biofabrication. For significant results, antibacterial assessment of human pathogens S. aureus and E. coli is performed using standard disc diffusion. The biosynthesis of silver nanoparticles and their properties toward the bacterial pathogen V. cholera are also assessed. While antibacterial agents are used, changes in membrane porousness and the reciprocal silver NP exposure in bacterial cells have been reported [ 111 ].
Antibacterial activities of Ag nanoparticles interacting with sodium alginate films are exposed to S. aureus strains, and disc diffusion depicts an antibacterial potential toward bacteria. The antibacterial coating is implemented on the pear and carrot exteriors, and the outcome is differentiating between exposed and unexposed samples. The silver nanoparticles significantly break the polymer subgroups of the cell wall in disease-causing bacteria. The considerable role of NPs frequently damages the cell barrier and breaks protein pathways in bacteria. The higher ratios of Ag nanoparticles cause quicker membrane damage than the low ratios and significantly damage the cell membrane of bacteria.
The Rhizophora apiculata -stabilized Ag nanoparticles reveal a lower ratio of bacterial growth on the culture plate compared to silver-nitrate-exposed cells; this might be because of the lower size of the materials and higher exterior interaction, which results in increased membrane damage and cell breakage in bacterial cells [ 112 ].
4.2. Fungicidal Activity
General antifungal agents can generate several side-effects such as diarrhea, increased renal failure, nausea, and increased body temperature; hence, for fungal diseases, substitutive treatment is needed. A recent study stated that Pt nanoparticles exhibited antifungal activity against several hazardous fungi such as C. acutatum , D. bryoniae , C. fulvum , P. capsici, and P. drechsleri . Biopolymer-based Pt nanocomposites GKPt NPs are tested for antifungal activity against various strains of fungi, such as A. parasiticus and A. flavus. Prior research has stated that the antifungal properties of Pt nanoparticles in a nano-mixture-induced membrane breakage raised the ratio of ROS, changed the shape of the mycelia, and resulted in damage to DNA and cellular breakage ( Figure 4 ) [ 113 ].
The fungicidal activity mechanism of biosynthesized metallic NPs has a higher efficiency compared to commonly available antibiotics such as amphotericin and fluconazole. The plant-extract-procured silver NPs have effectively revealed membrane breakage in Candida sp., which interrupts fungal intracellular constituents and results in cellular damage [ 114 ]. Some of the available antifungal substances have restricted implementation and also minimized activities and the lack of curing of microbial infections. The wide-spectrum properties of silver nanoparticles have an interesting activity toward spore-spreading fungus and significantly damage fungal development. Exposure to NPs effectively altered the fungal cell wall structure.
4.3. Anti-Plasmodial Activity
Recently, the main factors that generate disease are spreading ubiquitously through trajectories. Trajectory management is a crucial need in the current state. Further, the developed anti-plasmodial species’ unique management method has a higher cost and lower efficiency to manage the specific organism in the medical field. However, efficient and predominant antimalarial medicines remain needed to manage plasmodial properties. In previous years, plants were used for customary agents of usual outcome and had all the substances for drug manufacturing for antimalarial disease. Secondary phytochemical components such as artemisinin and quinine constituents have been effectively used against the resistant malaria parasite. The substitute drug was required for managing the various strains based on increased parasite defense. The plant extract produced metallic NPs such as Ag, Pt, and Pd NPs, which help control malarial growth. The bio-mediated amalgamation of metallic Ag NPs by plant extract has halted malarial growth [ 115 ].
4.4. Antiviral Activity
Antivirals have been studied in conjunction with different degrees of completion for Hepatitis C, and target-acting drugs have resulted in a higher 90% treatment ratio. Despite the better outcome revealed with Hepatitis C, target-acting antiviral technology was not followed, to induce activity due to limitations in availability [ 116 ]. Several viral infections, including specific virus targets, have been left unfinished due to the urgent need for antiviral drug resistance. Current research has found a link between pharmaceuticals and a specific viral target. Furthermore, developing technologies that interact with altered host components can induce a viral disease cure. One feasible target was host cell agents that are needed for viral duplication but are excreted by the host. These specificities reduce viral growth via termination duplication and also lower the activities of the host [ 117 ]. The inherent viral infections were the first line of defense against viral dysfunction and death. The secondary host was targeted to induce the immune action that led to tissue damage in the reaction of viral elimination. The host immune response may be another viable target for infection cure, to induce host-mediated viral control while restricting tissue-breaking immunopathology. Furthermore, antiviral therapy may stimulate collaboration with target-acting antivirals with the goal of hosting immuno-manipulation to clear up both anguish and death etiologies.
Plant-associated NPs serve as replacement drugs in the treatment and management of viral diseases. The virus’s entry into the host is extremely dangerous, and it requires a faster adaptation reaction to develop its growth. The biosynthesis of silver nanoparticles might play an efficient role as wide-scope antiviral substances to limit virus cell features. Ambrose et al. (2022) stated that the bio-mediated synthesized silver nanoparticles have effective anti-HIV agents at the prior step of the backward transcription mechanism [ 118 ]. Nanomaterials have effective antiviral substances that suppress the virus before it enters the host system. The bio-mediated synthesized metallic NPs have various coupling actions to enable them to interact with groups of viral cells to manage the features of viruses. The bio-associated NPs play a strong broad-spectrum agent role against cell-free viruses and cell-mediated viruses. Furthermore, Ag and Au NPs strongly suppress the HIV-1 life cycle before arrival. Furthermore, metallic nanoparticles have antiviral properties against retroviruses [ 118 ].
The antivirus pathway of magnesium nanoparticles based on their metal ions, size, and shape—especially stabilized magnesium nanoparticles—reveals a notably higher number of connections, including those between host cells and viruses differentiated with nanoparticles. The antivirus process for MNPs can take place inside or outside of the host cell [ 119 ]. The mechanism, which began when nanoparticles communicated with gp120 proteins, terminated host cell coupling positions and suppressed virus adhesion to host cells ( Figure 4 ). Another feasible mechanism was mediated by scattering the virus pieces before they made their way into the cell, which caused viral genome coupling to the virus pieces. The MONPs might be applied as antivirus agents by coupling the materials to the exterior of the virus [ 120 ]. This holds the connections between the coupling area on the exterior of the virus and the receivers on the exterior of the host cell. Furthermore, the virus does not enter the cell. MNPs, particularly silver and gold nanoparticles, are said to have antiviral properties against various viruses. Gold nanoparticles terminate the gp120 coupling to CD4 and suppress the virus’s arrival, while silver nanoparticles suppress viral arrival, coupling, and growth. Silver NPs terminate CD4-associated virion coupling, amalgamation, and pathogenesis by connecting with the viral gp120 in the cell-mediated viruses. In dual-standard viruses, the silver NPs, after connecting with the viral genome, terminate virus growth. Zn nanoparticles disturb viral DNA polymerase activity, resulting in the termination of viral growth. The size of Zn nanoparticles combined with virions may inhibit virus entry into the cell.
4.5. Anti-Inflammatory Activity
Anti-inflammatory therapy is a cascade method that induces immune reactive composites such as cytokinins and interleukins that may develop keratinocytes as well as T, B, and C lymphocytes and macrophages. The endocrine system produces several anti-inflammatory mediators, such as antibiotics and enzymes. Another significant anti-inflammatory substance, such as cytokines (IL-1 and IL-2), is formed via the primary immune organs. These anti-inflammatory agents stimulate healing activity. Inflammatory arbitrators occur in biochemical mechanisms and control disease spread. Bio-mediated, obtained AgNPs attain a better wound healing pathway and tissue regrowth in inflammatory features. According to one study, bio-mediated Au and Pt NPs are substitute agents for treating inflammation via the traditional route [ 121 ].
In recent years, nanoparticles have been developed as anti-inflammatory agents. Nanoparticles have a high exterior-position-to-interior ratio and are used to block inflammatory agents such as cytokines and inflammation-mediating enzymes, as well as other supplements. Several metal-mediated nanoparticles, such as those based on Ag, Au, copper, and iron oxide, have been reported to have effective anti-inflammatory properties. Swelling is the body’s immediate response to internal breakage, transmission, hormone checks, and damage in the internal shape and outer functions, such as infection by infectious microorganisms or an external component. Individual resistance cells trigger antigen receptors to assume biochemical actions. Inflammation is affected by tissue and cellular damage, which leads to a disparity in the signals managing the inflammation. When injured or infected, tissue produces an inflammatory response that results in the formation of macrophages and killer cells. Macrophages have an important role in auto-inflammatory reactions. Macrophages are large, single-nucleated phagocytes that develop in the bone marrow as completed white blood cells migrate to monocytes in the bloodstream. These monocytes then float to various tissues and develop into macrophages. Macrophages are divided into two steps: pro-inflammatory M1 macrophages, whose development induces inflammation, and anti-inflammatory M2 macrophages, which are activated as an anti-inflammatory reaction and induce the reassembly of the inflamed tissue and organs. Macrophages are capable of withstanding the inflammation reaction by stimulating the two characteristics unexpected in the retarder’s disorder. The macrophages overcome the inflammation and skin injury via phagocytosis, resulting in inflammation via initiation signals inducing the macrophages [ 122 ].
4.6. Antidiabetic Activity
A type of metabolic disorder in which the blood sugar ratio is uncontrolled is diabetes mellitus. Some foods and stability diets, as well as synthetic drugs, may suppress diabetes in some food scales, making DM therapy a difficult task. Furthermore, the biosynthesized NPs could be used as a replacement drug to treat diabetes mellitus. According to Daisy and Saipriya (2012), Au NPs have better medicinal activity for diabetic control. Au nanoparticles effectively lower the ratio of liver enzymes such as alanine movement, serum creatinine, uric acid alkaline, and phosphatase in exposed diabetes mice. Au NPs exposed to a diabetic model demonstrated a reduction in the HbA (glycosylated hemoglobin) scale that was managing the standard scale [ 123 ]. Swarnalatha et al.’s (2012) research stated that Sphaeranthus amaranthoides -bio-medially obtained AgNPs suppressed a-amylase and a carbohydrate sugar in diabetes under an animal study [ 124 ]. Premna herbacea Roxb extract contains antidiabetic properties [ 125 ]. Pickup et al. (2008) stated that NPs are important treatment substances for better diabetes management. The medicinal research in mice completely managed the sugar ratio of 140 mg/dL in Ag NP treatment [ 126 ].
4.7. Antioxidant Activity
Antioxidant activity, along with non-enzymatic and enzymatic agents, manages free radical development. Along with brain injury, atherosclerosis, and cancer, free radicals target cellular breakage. Free radicals are developed through ROS such as hydrogen peroxide and SOD. Bio-constituents such as glycoproteins, proteins, phenolics, lipids, and flavonoids effectively manage free radical development. Furthermore, the scavenging action of antioxidants is essential to controlling several diseases, such as neurodegenerative diseases and metabolic diseases. Silver nanoparticles have stronger antioxidant properties compared to standard drugs such as ascorbic acid.
The nanoparticles revealed increased antioxidant activity, and the tea extract showed increased flavonoids and phenolic components. Yazdi et al. (2020), stated that reactive oxygen species and free radicals have revealed activity in the biological system [ 127 ]. These agents are natural metabolic outcomes that damage cell development, which leads to cell breakage, the unreliability of biological components, and damage to common features in several cells.
Oxidative stress is involved in the epidemic of several diseases, including cancer, Alzheimer’s disease, and blindness. Plants have a lot of antioxidants, which protect human health because they strongly preserve biological systems against these substances, select toxic free radicals, and lower cell destruction. The nanomaterial is familiar as a vehicle system for selected drug transmission in current years [ 128 ]. The CeO 2 nanoparticles used as vehicles for cancer therapy due to their antioxidant activities might standardize ROS. Current research states that these nanoparticles have anti-cancer activities during their antioxidant activities and secure healthy cells [ 129 ]. Bio-mediated synthesized nanoparticles revealed various biomedical applications, which are depicted in Table 6 .
Various source-synthesized nanoparticles used for biomedical application.
4.8. Anticancer Therapy
Cancer is one of the deadliest causes of death today [ 150 ]. Cancer develops due to environmental and genetic factors. Several advancements due to the use of different nanoparticles have been made in recent years. Some of the nanoparticles are highlighted in this section. A silver nanoparticle obtained from the probiotic bacteria L. rhamnosus GG was very helpful in dealing with HT-29 cells in colorectal cancer [ 150 ]. Gold nanoparticles from L. kimchicus DCY51T showed excellent anticancer activity against A549 cells (a human lung adenocarcinoma cell line) and HT29 cells (a human colorectal adenocarcinoma cell line) [ 150 ]. SeNPs from L. casei were very effective against colon cancer cells. CuO NPs obtained from L. casei subsp. casei were found to be effective against human gastric carcinoma cells (AGS) and the human colon carcinoma cell line (HT-29) [ 150 ]. Pt nanoparticles obtained from Streptomyces sp. were found to be effective against the breast cancer MCF-7 cell line [ 150 ]. Furthermore, ZnO obtained from Lactobacillus spp. was found to be effective against human colon cancer (HT-29) [ 150 ]. Thus, it is clear that different nanoparticles obtained from the biosynthesis approach have effective actions toward cancer treatment.
4.9. Bio-Sensing Applications
The sensing of biological materials using nanoparticles is very useful for the betterment of mankind [ 151 ]. Several nanoparticles are used for these kinds of bio-sensing applications [ 152 ]. In a study, chloroplast-mediated green synthesis of Au-Ag alloy was used to analyze cancer [ 153 ]. S. myriocystum -mediated PtNPs were used for the detection of allergies and asthma [ 154 ]. Hypnea valencia -mediated synthesis of AuNPs was used to detect pregnancy in women [ 155 ]. Furthermore, Noctiluca scintillans -mediated biosynthesis of AgNPs was evaluated to detect mouth gum and oral discharge issues [ 156 ].
4.10. Other Medical Applications
The wound-healing properties of silver NPs obtained by the extracellular method from Aspergillus niger were studied using a rat model for an ablation and thermal wound. The studies reported that silver NPs have effective antimicrobial properties and that nano-Ag can control cytokines that occur during wound restoration. Other medical implementation studies with diabetes, especially the suppression of the enzyme PTP, class PTP1B, Interrupting PTP is a cause of various diseases, such as cancer and metabolic diseases [ 157 ]. A quick formation of gold nanoparticles containing guavanoic acid via Psidium guajava leaf extract was observed. These were applied in the PTP-1B suppressive study, which revealed the importance of suppressive action with an IC 50 of 1.14 g/mL −1 . The biofabrication of gold nanoparticles and their implementation on the glucose biosensor for blood glucose identification were reported by Zheng et al. (2022) [ 158 ]. The biosensor is used to determine glucose levels in blood samples, revealing the distribution along with commercial clinical protocols. Other research reported that the green medium mediated the obtaining of gold and silver nanoparticles and was reduced by Brevibacteriumcasei . These nanoparticles revealed anti-coagulant properties by suppressing the development of blood clots in the sample that attained blood, including silver nanoparticles [ 159 ]. The solidity of Au and Ag nanoparticles in the blood was also confirmed through the treatment of the blood plasma with the materials for 1 day, which did not reveal any considerable lowering in the properties [ 160 ].
5. Conclusions and Future Scope
The nanotechnology field is mainly interconnected with physics, chemistry, biology, and material science, and it creates new biomedical nano-sized particles for therapeutic and pharmaceutical implementations. In recent years, nanoscience has gained researchers’ interest because of its significant applications in medical diagnosis, pharmacy, disease curing, electronics, agriculture, space, and chemical industries. Nanoparticles are currently thought to be extremely useful materials. The biologically mediated nanoparticles are procured through various organisms such as plants, bacteria, fungi, actinomycetes, and yeast. Bio-mediated nanoparticles have been widely used to treat various pathogenic diseases while having fewer toxic effects. Non-biological approaches such as physical and chemical methods are used in the production of nanoparticles, which have a variety of toxic properties for the environment and human health. Hence, as a result, biologically mediated metallic nanoparticles are non-toxic, less expensive, and less harmful to the environment. Moreover, the obtained nanoparticles might stimulate activity using genetic engineering methods. Bio-mediated nanoparticles have specific properties such as being more biocompatible, having a larger surface area, being more reactive, and being non-toxic. The current review provides a clear point of view on various sources of synthesized nanoparticles and their medical applications, such as antibacterial, antifungal, antiviral, anti-inflammatory, antidiabetic, antioxidant, and other medical applications.
Acknowledgments
E.-B. Cho and Gopalu Karunakaran were supported by Brain Pool Program through the National Research Foundation of Korea (NRF) funded by the Ministry of Science and ICT (Grant no. 2022H1D3A2A02044281).
Funding Statement
E.-B. Cho and Gopalu Karunakaran were supported by the Brain Pool Program through the National Research Foundation of Korea (NRF) funded by the Ministry of Science and ICT (Grant No. 2022H1D3A2A02044281).
Author Contributions
Conceptualization, G.K. and K.G.S.; methodology, G.K. and K.G.S.; resources, E.-B.C.; writing—original draft preparation, G.K. and K.G.S.; writing—review and editing, S.A. and E.-B.C.; visualization. All authors have read and agreed to the published version of the manuscript.
Institutional Review Board Statement
Informed consent statement, data availability statement, conflicts of interest.
The authors declare no conflict of interest.
Sample Availability
Disclaimer/Publisher’s Note: The statements, opinions and data contained in all publications are solely those of the individual author(s) and contributor(s) and not of MDPI and/or the editor(s). MDPI and/or the editor(s) disclaim responsibility for any injury to people or property resulting from any ideas, methods, instructions or products referred to in the content.
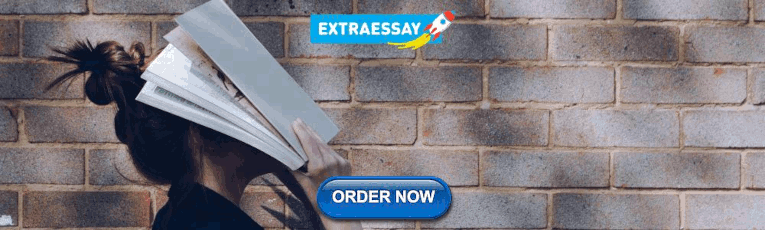
IMAGES
VIDEO
COMMENTS
Introduction. Nanoscience has gained remarkable attention due to its diverse applications in multiple fields. The cornerstone of nanoscience is the cost-effective synthesis of nanoparticles (NPs) with unique physical, chemical, optical, electrochemical, and thermal properties [1, 2].The applications of NPs in the environment sector have good potential to overcome the challenges of ...
Green synthesis method; provides a faster metallic nanoparticle production by offering an environmentally friendly, simple, economical and reproducible approach. Given the wide range of ...
Green synthesis of Au NPs, NZVI, iron oxide nanoparticles, and Cu NPs has also tried to make full use of local plants. Fenugreek, used in the synthesis of Au NPs, is widely distributed in China and the east coast of the Mediterranean, while peppermint is native to Central Europe and West Asia ( Aswathy Aromal and Philip, 2012 ).
Green synthesis of nanoparticles has many potential applications in environmental and biomedical fields. Green synthesis aims in particular at decreasing the usage of toxic chemicals. For instance, the use of biological materials such as plants is usually safe. Plants also contain reducing and capping agents. Here we present the principles of green chemistry, and we review plant-mediated ...
In the past few years, nanoparticles have been applied in various fields of science and technology, ranging from material science to biotechnology. Thus, the synthesis of nanoparticles can be considered as a dynamic area in research and application of nanoparticles. The different methods of nanoparticle synthesis include physical, chemical, and biological methods. Of these methods, the ...
We also provide an overview of the biomolecules that were found to be suitable for NP synthesis. This work is meant to be a support for researchers who intend to develop new green approaches for the synthesis of NPs. Keywords: metal nanoparticle; metal oxide nanoparticle; green synthesis; biological method. 1.
peel extract. After addition of the reducing agent the gradual generation of silver nanoparticles. started which is indicated by the increase in the absorbance intensity at 400-410nm in UV. spectra.The Orange peel extract showing absorption bands at 324 and around 284 nm Figure 3.
In the arena of material sciences, one of the burning topics for research has been biogenically synthesizing nanoparticles (NPs) from plant derivatives and studying their applicability to be used as sustainable catalysts. The contemporary work happening on nanocatalysts focuses on the scope of application of green catalysts. For devising a technology that is ecofriendly as well as rapid, it ...
Green synthesis of nanoparticles (NPs) using plant materials and microorganisms has evolved as a sustainable alternative to conventional techniques that rely on toxic chemicals. Recently, green-synthesized eco-friendly NPs have attracted inter - ... thesis, potential mechanisms, and prospective applications of NPs generated by biological factories.
Green synthesis of metal nanoparticles and assessment of their biological activity: Researcher: Prachi: Guide(s): Negi, D.S. Keywords: Biological Activity Chemistry Chemistry Multidisciplinary Green Synthesis Metal Nanoparticles Physical Sciences: University: Hemwati Nandan Bahuguna Garhwal University: Completed Date: 2019: Abstract:
The use of plants for green nanoparticle synthesis is an interesting and emerging aspect of nanotechnology that has a significant impact on the environment and contributes to nanoscience's long-term sustainability and progress. Catalysis, medicine, cosmetic, agriculture, food packaging, water treatment, dye degradation, textile engineering ...
The physical process involves laser ablation, condensation, evaporation, etc., whereas the chemical process involves hydrazine, sodium borohydride, green synthesis, etc. Using plant species to produce nanoparticles has been termed a green technique ( Figure 2 and Figure 3) and the most reliable environmentally sustainable approach [ 24, 25 ].
Nanotechnology is the branch of science which deals with the examination of materials in nanorange, generally between 1 to 100 nm. It is a science that works at the nanoscale and gives various focal points to the diverse fields of science like dentistry, pharmaceuticals and bio-engineering [ Citation 1 ]. Green chemistry approach is significant ...
4.1. Block copolymers with polylactides (PLA) PLA is a thermoplastic, recyclable artificial fiber made from inexhaustible ingredients such as maize starch or sugarcane. PLA's profile similarity and biodegradability make it ideal for sedate conveyance, tissue design, and nanoparticles modification.
Synthesis of green and pure copper oxide nanoparticles using two plant resources via solid-state route and their phytotoxicity assessment. Iman Khaldari a, Mohammad Reza Naghavi * a and Elaheh Motamedi * b a Division of Biotechnology, Department of Agronomy and Plant Breeding, Agricultural and Natural Resources College, University of Tehran, Karaj, Iran. E-mail: [email protected] b Department ...
A scale covering 1-100 nm. 8. Nanomaterial. A material is called a nanomaterial if it has at least one dimension in the nanoscale range of 1-100 nm. 19. Nano-object. A nano-object is a discrete piece of material with one, two, or three external dimensions in the nanoscale range. 3 and 20. Nanoparticle.
Selenium nanoparticles (SeNPs) are extremely popular objects in nanotechnology. "Green" synthesis has special advantages due to the growing necessity for environmentally friendly, non-toxic, and low-cost methods. This review considers the biosynthesis mechanism of bacteria, fungi, algae, and plants, including the role of various biological substances in the processes of reducing selenium ...
Nanoparticles are often associated with their small size and numerous applications. However, the synthesis process is equally important as it determines the size and properties of the nanoparticles. While traditional nanoparticle synthesis methods require the use of hazardous chemicals and high-energy consumption, green synthesis offers a sustainable, cost-effective, and environmentally ...
nanoparticles (NP), and their application on pathogenic bacteria were investigated. ZnO NP were synthesized by chemical reduction method using starch as capping agent and silver NP was prepared by green synthesis process from AgNO3 solution through the extract of Citrus sinensis (sweet lime). The detail characterization of the nanoparticles was ...
The synthesis of diverse metal nanoparticles utilizing bioactive agents, including plant materials, microbes, and various biowastes like vegetable waste, fruit peel waste, eggshell, agricultural waste, algae, and so on, is known as "green" or "biological" nanoparticle synthesis (Kumari et al., 2021).
Green-mediated nanoparticle synthesis is a low-cost, environmentally friendly method with no toxic properties. This method uses various stabilizing and reducing substances, such as plants, microbes, and some natural agents, to develop NPs. The green-mediated obtaining of nanoparticles has gained popularity due to their low cost, non-toxicity ...