Thank you for visiting nature.com. You are using a browser version with limited support for CSS. To obtain the best experience, we recommend you use a more up to date browser (or turn off compatibility mode in Internet Explorer). In the meantime, to ensure continued support, we are displaying the site without styles and JavaScript.
- View all journals
- Explore content
- About the journal
- Publish with us
- Sign up for alerts
- News & Views
- Published: 16 January 2023
- Hydroelectricity
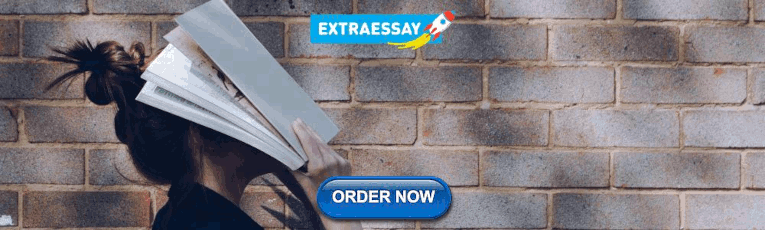
The potential for sustainable hydropower
- Patrick Bogaart ORCID: orcid.org/0000-0002-8612-1289 1
Nature Water volume 1 , pages 22–23 ( 2023 ) Cite this article
4593 Accesses
5 Citations
31 Altmetric
Metrics details
- Environmental impact
Development of the world’s hydropower is controversial because of its many documented environmental and societal implications. A global assessment of the unused profitable hydropower potential is performed with strict criteria to limit the environmental and social impact.
This is a preview of subscription content, access via your institution
Access options
Subscribe to this journal
Receive 12 digital issues and online access to articles
92,52 € per year
only 7,71 € per issue
Buy this article
- Purchase on Springer Link
- Instant access to full article PDF
Prices may be subject to local taxes which are calculated during checkout
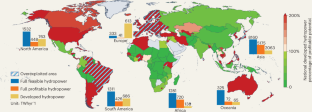
IPCC Climate Change 2022 : Summary for Policymakers(eds Shukla, P. R. et al.) (Cambridge University Press, 2022).
Hydroelectricity (IEA, 2022); https://www.iea.org/reports/hydroelectricity
Owen, J. R. et al. Nat. Sustain . https://doi.org/10.1038/s41893-022-00994-6 (2022).
Cash, D. W. et al. Proc. Natl. Acad. Sci. 100 , 8086–8091 (2003).
Article CAS Google Scholar
Xu, R. et al. Nat. Water https://doi.org/10.1038/s44221-022-00004-1 (2023).
Forsberg, B. R. et al. PLoS ONE 12 , e0182254 (2017).
Article Google Scholar
Moran, E. F., Lopez, M. C., Moore, N., Müller, N. & Hyndman, D. W. Proc. Natl. Acad. Sci . 115 , 11891–11898 (2018).
Best, J. Nat. Geosci . 12 , 7–21 (2019).
Gernaat, D. E. H. J. et al. Nat. Energy 2 , 821–828 (2017).
Download references
Author information
Authors and affiliations.
Statistics Netherlands, The Hague, Netherlands
Patrick Bogaart
You can also search for this author in PubMed Google Scholar
Corresponding author
Correspondence to Patrick Bogaart .
Rights and permissions
Reprints and permissions
About this article
Cite this article.
Bogaart, P. The potential for sustainable hydropower. Nat Water 1 , 22–23 (2023). https://doi.org/10.1038/s44221-022-00018-9
Download citation
Published : 16 January 2023
Issue Date : January 2023
DOI : https://doi.org/10.1038/s44221-022-00018-9
Share this article
Anyone you share the following link with will be able to read this content:
Sorry, a shareable link is not currently available for this article.
Provided by the Springer Nature SharedIt content-sharing initiative
Quick links
- Explore articles by subject
- Guide to authors
- Editorial policies
Sign up for the Nature Briefing: Anthropocene newsletter — what matters in anthropocene research, free to your inbox weekly.

- Reference Manager
- Simple TEXT file
People also looked at
Original research article, the effect of precipitation on hydropower generation capacity: a perspective of climate change.

- 1 Ministry of Education Key Laboratory of Resources and Environmental Systems Optimization, North China Electric Power University, Beijing, China
- 2 The Electricity Engineering School, Guangxi University, Nanning, China
- 3 State Key Laboratory of Simulation and Regulation of Water Cycle in River Basin, China Institute of Water Resources and Hydropower Research, Beijing, China
Hydropower, which is the most extensively used renewable energy, is sensitive to the change of streamflow under the great impact of precipitation. According to the relationship between the hydropower station generation and local precipitation, the impact of precipitation on hydropower can be analyzed. In this paper, the global climate model and regional climate model simulations in precipitation are compared firstly, and the high-resolution precipitation data are then calculated by stepwise clustering analysis statistical downscaling method. Secondly, based on the hydropower potential (HP), the hydropower response model driven by precipitation is established. Finally, the simulated generation of a hydropower station in Dadu River basin is used as a case to validate this proposed model. The results show that precipitation will increase by around 42% from May to August in study region, while it will decrease by 40% in other months in RCP4.5. For different periods of reservoir scheduling, the precipitation will increase by about 40% in the Neutral I and Wet period, while it will decrease by around 30% in other periods, which will lead to the shortening of the peak period of hydropower generation and the peak value will be decreased. Correspondingly, the results show power generation will decrease by around 12% from June to December and increase by around 4% in the rest months. On the other hand, owing to the changes in precipitation, the future power generation will increase by 25% in Neutral I and decrease by 13.5% in other periods, but the total hydropower generation will remain. The results can provide some decision support for future water resources management in Dadu river basin, especially for the planning and operation of hydropower stations.
Introduction
According to the IPCC AR5, with the increase of global populations and economies, the man-made emissions of greenhouse gases have remained rising and reached the industrial history peak at the beginning of the 21st century ( IPCC, 2013 ). The global mean temperature at the end of the 21st century will probably be 1.5°C higher than that in 1899–1990 under the influence of current emission trends ( Arnette, 2017 ).
China, which is located in East Asia, has a vast territory, abundant climate zones and complex topography ( Guo et al., 2017a ). Owing to the stronger regional seasonal wind led by global warming, more moisture in the atmosphere will be transported to land areas from ocean, then resulting in more extreme precipitation events in China recently ( Hui et al., 2018 ). For example, ten million residents were displaced and billion dollars economy cost was caused by the Yangtze River flood in 1998 ( Chen and Zong, 2000 ). The serious urban waterlogging events in Beijing in 2012 caused thousands people death and 11.6 billion economic losses ( Zhang D.L. et al., 2013 ).
As one of the effective energies to alleviate the greenhouse effect caused by fossil energy, renewable energy is in a fast-growing demand. Renewable energy has taken 18.2% in world total energy consumption in 2016 ( REN21, 2018 ). In the meantime, the development of renewable energy in China is also rapidly developed. Renewable energy generation, which is up to 1676 TWh, has taken 30% in total national power generation in 2017 ( CREO, 2018 ). Relative to other types of renewable energy, owing to the lowest cost, easier to control and manage and lower intermittent and uncertainly means, hydropower is a kind of the most widely used renewable energies ( Panwar et al., 2011 ; Chu and Majumdar, 2012 ; Jiang et al., 2018 ). In 2017, the new generating capacity of hydropower in China is 9.12 GW, and the total installed capacity reached 341 GW. The hydropower generation has taken almost 20% of total power generation, which is far more than the generation of wind energy (5%) and solar energy (2%). Therefore, more hydropower is planned by China’s government to generate electricity and replace fuel energy ( IHA, 2018 ).
Hydropower is sensitive to climate change, especially for precipitation. Based on the change of runoff, Ali et al. (2018) evaluated and predicted the power generation of seven large hydropower stations in India. Results illustrated that the specific future climate may increase hydropower generation by about 25%. Liu et al. (2016) thought runoff and reservoir storage has a great influence on hydropower, then Generation Hydropower Potential (GHP) and Development Hydropower Potential (DHP) are tested based on 8 Global Hydro Models (GHMs), indicating that GHP will have a 3–6% increase between 2070 and 2099. Obviously, precipitation has a direct influence on basin runoff change, which will finally affect hydropower generation ( Berghuijs et al., 2014 ; Wasko and Sharma, 2017 ). Based on the observed data, Chilkoti et al. (2017) found that generation of hydropower stations and the precipitation have close links, the generation of hydropower stations may rise 39% when the precipitation rise 43%. Above all, climate change has a more direct impact on precipitation, but few studies are analyzing the influence of precipitation changes under the context of global warming on hydropower generation ( Guo et al., 2018 ). Hence, it is significant to explore the relationship between precipitation changes and hydropower generation, and the results could provide some suggestions and measures to the government policy makers in hydropower to response the climate change better.
In this study, stepwise cluster analysis (SCA) statistic method is used to downscale future climate data from PRECIS regional climate model (RCM). Then, the hydropower response model driven by precipitation (HRMDP) is established. Finally, a hydropower station in Dadu River basin is taken as a case to quantitatively analyze future climate change influence on hydropower generation.
Data and Methods
As shown in the Figure 1 , four analysis steps are divided in this paper. The first step is collecting precipitation and power generation data, which include large-scale HadGEM2-ES climate data, observation of meteorological stations and generation data of hydropower stations. Then, the statistical downscaling simulation in precipitation using SCA method is conducted. Specifically, some independent variables in large scale grids in PRECIS RCM, such as precipitation, temperature, humidity and pressure are extracted firstly, afterward, the precipitation in station scale is simulated and projected by the SCA statistic downscaling method. The third step is the establishment of a HRMDP based on the hydropower potential (HP). In the end, we use the projected precipitation under RCP4.5 and RCP8.5 climate emission scenarios to drive the HRMDP to analyze the change in hydropower in the case.
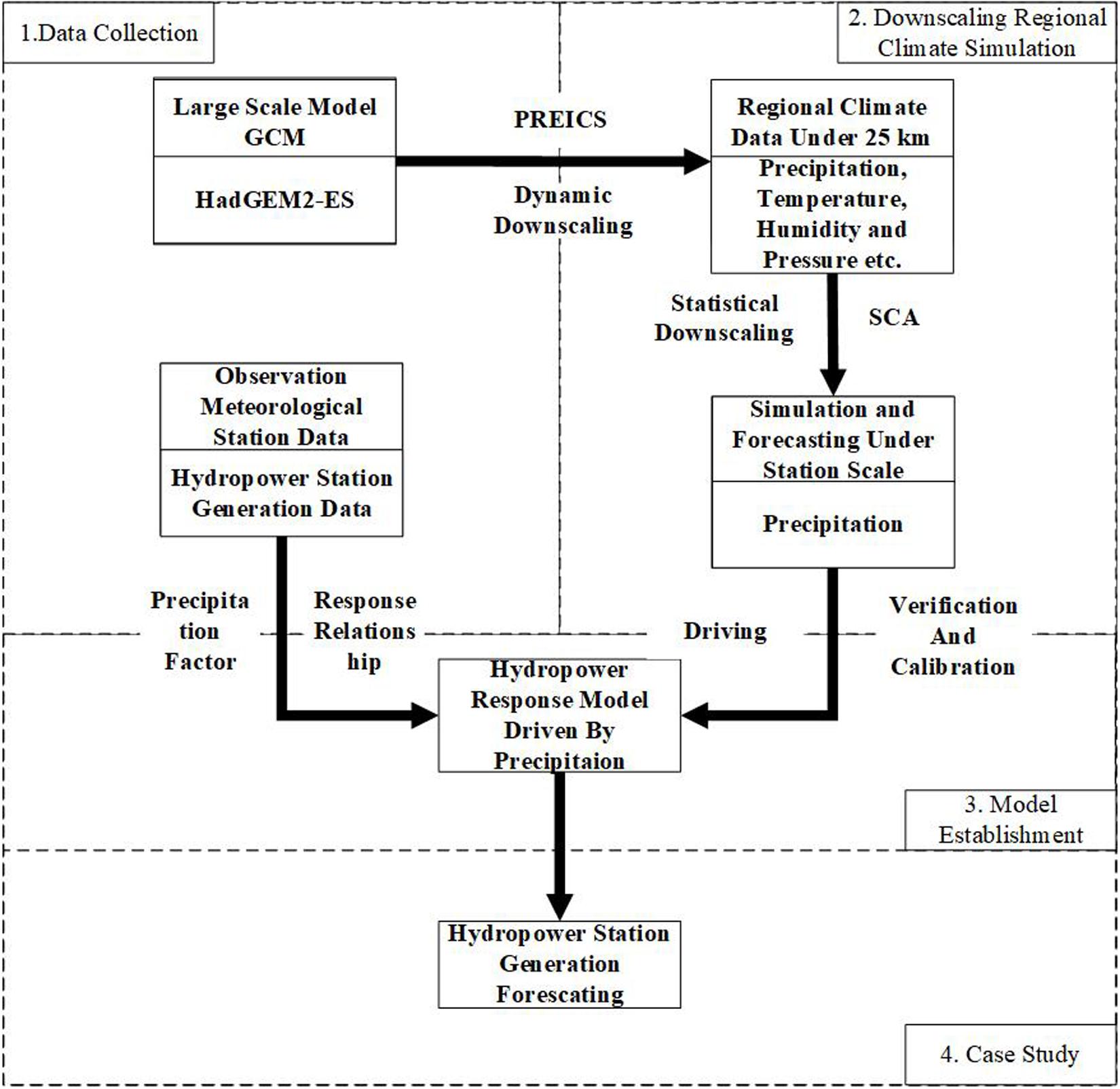
Figure 1. Study flow chart.
Hydropower Response Model Driven by Precipitation
In general, hydropower generation has a close relationship to precipitation. The increase in precipitation will lead to an increase in power generation, and continuous non-precipitation will cause a decrease in power generation ( Chilkoti et al., 2017 ). Figure 2 shows that the daily precipitation and daily power generation of station A in Dadu River basin. The high power generation can be found in a high precipitation period (such as from June to September), while low power generation in low precipitation periods (such as from January to April and December). At the same period, power generation shows an increasing trend with increasing precipitation, and vice versa. According to Figure 2 , we can find that there is a certain relationship between precipitation and power generation, and the power generation is sensitive to the change of precipitation.
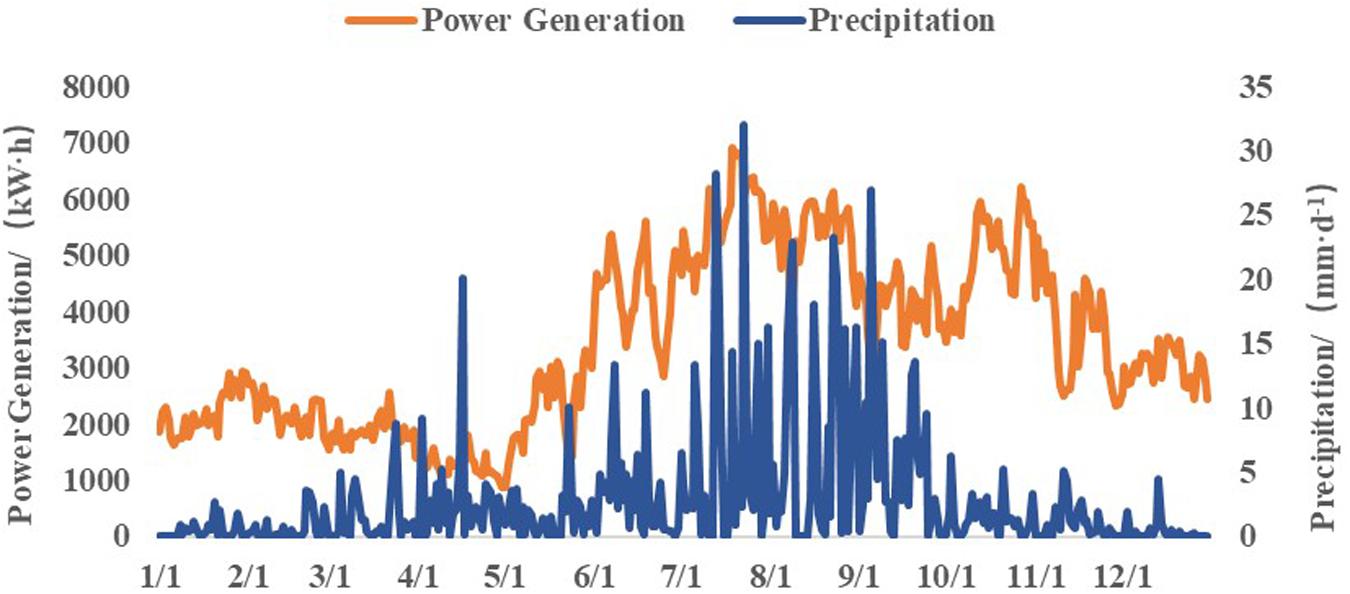
Figure 2. Daily precipitation and power generation of a hydropower station in Dadu River basin.
Before establishing the hydropower response model, a new conception – HP is introduced. Hydropower potential is the perfect state power generation which only considers the precipitation as the influence condition, it can be calculated by daily precipitation without considering the actual generating capacity. In this paper, HP mainly depends on the daily precipitation. In addition, continually precipitation factor and non-precipitation factor also have influence on HP, which respectively represents the increased coefficient of power generation in the period of continue precipitation and the decline coefficient of power generation in the period of non-precipitation. The detailed calculation method is as follows:
HP n – HP in the day n , kWh; R n – Precipitation in the day n , mm; σ 1 – The increasing coefficient of generation in the period of continue precipitation, dimensionless; σ 2 – The decline coefficient of generation in the period of non-precipitation, dimensionless; P n – The power generation in the day n , kWh; j – The number of days in some periods.
Hydropower potential represents the optimal power generation under the current precipitation status. Station power generation is not only restricted by natural factors but also influenced by human society factors (such as power policies and plans). Hence, the controllable coefficient, which represents human characteristics, is added to the formula. Formula b is used to restrict the power generation of actual production, and finally achieves the amount of constrained power generation.
HP max – Maximum HP during this period, kWh; HP min – Minimum HP during this period, kWh; P max – Maximum power generation of generator set under the power plan of this period; P m in – Minimum power generation of generator set under the power plan of this period; P F,n – The constrained power generation in day n of the period; δ – The controllable factor, which affected by actual power plan, power policy and more, and it shows as power generation fluctuates at a certain range.
Regional Climate Model
The future precipitation forecasting data are from two climate numerical simulations, which includes HadGEM2-ES and PRECIS. The former is a global climate model (GCM) and is used as the initial and boundary field data to drive the RCM PRECIS for dynamic downscaling. Developed by the UK’s The Met Office Hadley Centre, PRECIS is a high-resolution (25 km) RCM and it has been widely used in regional climate simulations and extreme events forecasting ( Xu et al., 2009 ; Kerkhoff et al., 2014 ; Saini et al., 2015 ; Guo et al., 2017b , 2018 ; Hui et al., 2018 ).
SCA Statistical Downscaling Method
Although the results from RCMs can simulate the climate change of the whole region or basin, the higher-resolution simulations (i.e., station scale) still cannot be met through dynamical downscaling. Therefore, based on the results of RCM, statistical downscaling can be achieved by establishing the numerical statistical relation between the large-scale circulation fields and station-scale climate variables (i.e., precipitation). There are rich methods to be applied, the statistical downscaling has been widely applied in climate change influence analysis on precipitation and hydrology ( Bellouin et al., 2011 ; Jones et al., 2011 ; Wang et al., 2015 ). However, it is necessary to select an appropriate statistical downscaling method to reflect the discrete and random characteristics for precipitation. The SCA uses a cluster tree to illustrate the relationship between the large-scale atmosphere fields and high spatial-resolution variables, especially in effectively dealing with the stochastic and non-linear relationships. Through the integration of missing data detection, correlation analysis, model calibration, cluster tree mapping and other auxiliary function modules, SCA can rapidly develop downscaling scenarios of local weather variables under current and future climate forcing, and performs well in predicting precipitation during the verification ( Chen et al., 2011 ; Yang et al., 2016 ).
As shown in Figure 3 , the first step in the SCA statistical downscaling is to select large-scale forecast factors. Following previous studies, the variables, including mean sea level pressure, surface airflow strength, surface wind direction, near-surface temperature, surface specific humidity, surface relative humidity, surface divergence, surface meridional velocity and more are selected as initial variables ( Duan and Mei, 2013 ; Li and Yan, 2017 ). According to correlation analyze, Near-surface temperature, Surface relative humidity and Mean sea level pressure are selected as independent variables in statistical downscaling ( Wood et al., 2004 ; Perkins et al., 2007 ; Liu et al., 2011 ). The meteorological station precipitation data are selected as dependent variable. Then, the independent variables of statistical downscaling are constituted as X matrix. The dependent variable is constituted as Y matrix. And using R Studio to create site scale precipitation SCA statistical downscaling training model, and build the clustering tree predictor to establish the quantitative relationship between precipitation and large-scale prediction factors.
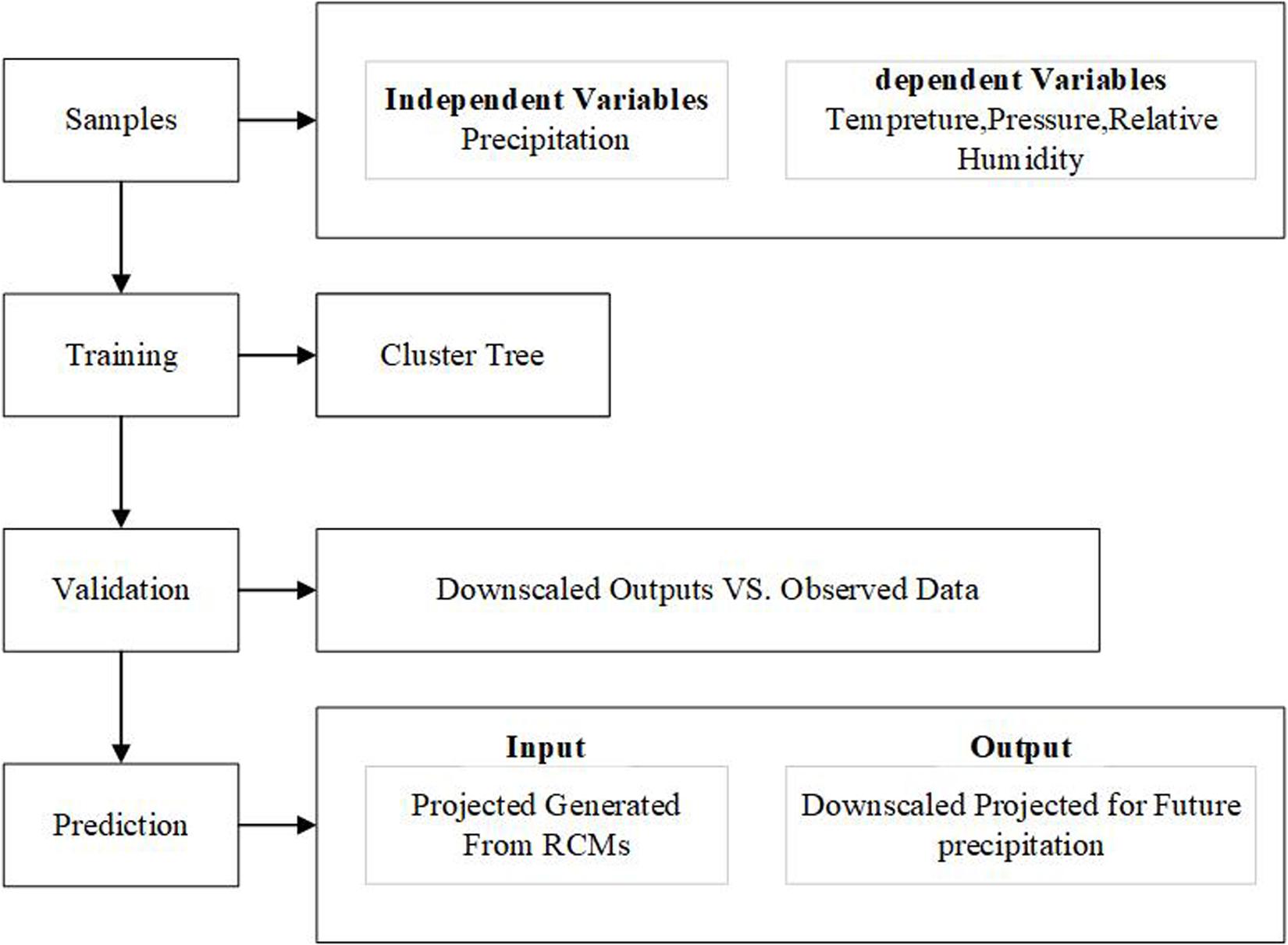
Figure 3. Stepwise cluster analysis downscaling flow chart.
The data used for validation is divided into the following categories:
1. Climate simulation data. The future climate data from PRECIS are used to drive the power forecasting model. Its initial field and boundary field data as the driving data is from HadGEM2-ES(GCM). HadGEM2-ES is a coupled AOGCM with atmospheric resolution of N96 (1.875° × 1.25°) with 38 vertical levels and an ocean resolution of 1° (increasing to 1/3° at the equator) and 40 vertical levels. HadGEM2-ES also represents interactive land and ocean carbon cycles and dynamic vegetation with an option to prescribe either atmospheric CO 2 concentrations or to prescribe anthropogenic CO 2 emissions and simulate CO 2 concentrations as described. An interactive tropospheric chemistry scheme is also included, which simulates the evolution of atmospheric composition and interactions with atmospheric aerosols. The model time step is 30 min (atmosphere and land) and 1 h (ocean) ( Jones et al., 2011 ). The PRECIS is able to run at two different horizontal resolutions: 0.44° × 0.44° (approximately 50 km × 50 km) and 0.22° × 0.22° (approximately 25 km × 25 km), with 19 atmospheric levels in the vertical using a hybrid coordinate system ( Guo et al., 2017b ).
2. Climate Observation data. The CN05 precipitation data set is used to validate the precipitation results of GCM and RCM. CN05 is a dataset consisting of daily mean, maximum, and minimum temperature on a 0.25 × 0.25° grid has been constructed over mainland China for the 45-years period of 1961–2005. CN05 is derived from interpolating observations from 751 stations distributed throughout the entire Chinese territory except Taiwan. The interpolation follows basically the same approach used in generating the CRU dataset, whereby a gridded climatology is calculated first, and then a gridded anomaly is added to obtain the final data ( Xu et al., 2009 ).
3. Meteorological station data. The observation is used to validate the results of RCM and build the HRMDP. It is from the real-time monitoring stations, including daily precipitation, near-surface temperature, surface relative humidity and surface wind speed.
4. Hydropower station data. The daily power generation data is used to calibrate and verify the simulation results of the power forecasting model.
The Dadu River basin which shown in Figure 4 , is located between 99°42’E–103°48’E and 28°15’N–33°33’N and lies in the transition area of Tibet Plateau and Sichuan Basin. Dadu River basin meets the Minjiang River in Leshan. It is the largest tributary of the Minjiang River and the secondary tributary of the Yangzi River. Dadu River Basin has an abundant hydro resource, which has 7.74 × 10 4 km 2 basin area and a total of 149 tributaries. The mainstream, which is 1062 km long and 48.8 km 3 annual net flow, and has 33730 MW water resource reserve. The annual precipitation of Dadu River Basin is about 800–1000 mm, and the precipitation from May to October takes up 80% of the whole year ( Yang et al., 2017 ). As a key hydropower development area in China, Dadu River basin has many large power stations.
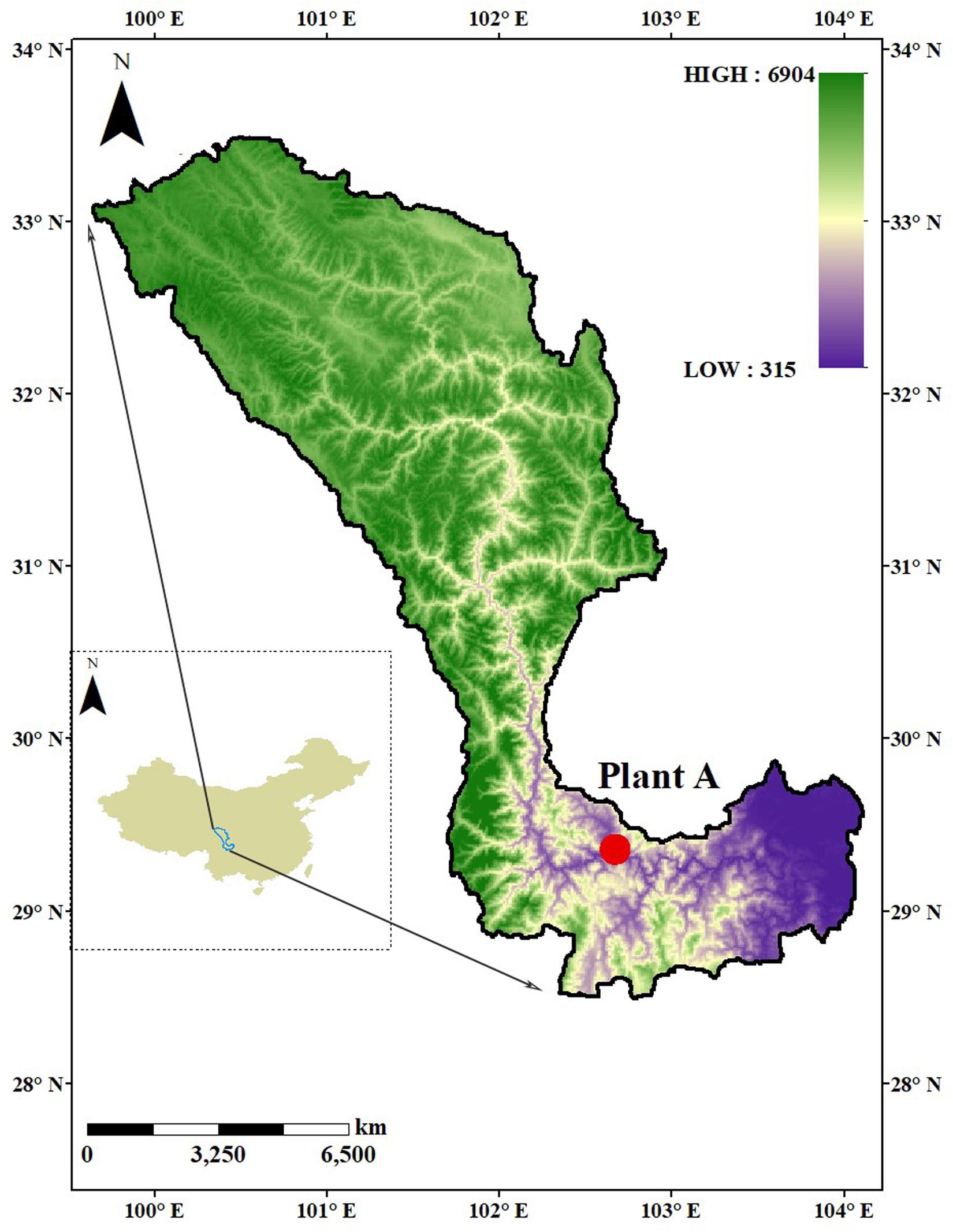
Figure 4. Dadu River basin.
Station A is one of the largest hydropower stations in China, the power capacity of station A is nearly 3.6 million KW, and the reservoir capacity is 5.337 billion km 3 . Due to the greatest power generation in Dadu River Basin and the most abundant water resources, station A is selected as the research object ( Zhang and Xu, 2014 ).
Moreover, according to the precipitation feature of Dadu River basin and power generation plan for station A. Five main power generation periods in a year are divided, which include Dry I (from January to April), Neutral I (May), Wet (from June to October), Neutral II (November) and Dry II (December).
In this paper, we take the power station A as a case and use HRMDP model to build the relationship between meteorological factors and power generation capacity. Then based on the projection in precipitation from dynamical and statistical downscaling results, the influence of precipitation on power generation from 2025 to 2035 will be analyzed.
Simulation and Verification
The results of climate models and their deviations with CN05 in different periods are shown in Figure 5 . Compared with the results of CN05, GCM underestimates the precipitation in most regions of the basin but overestimates the precipitation in the middle of basin. Due to the rough resolutions in GCM, the results of most areas only show a single value. On the other hand, PRECIS improves the spatial resolutions and shows more details in reproducing the precipitation in Dadu River basin, especially in autumn in Figure 6 . The results in PRECIS are similar to the observation, and the deviation is between -1 mm and 1 mm in the midstream. In terms of different periods, Dry II shows a better performance (±1 mm biases) than that in Dry I (∼2 mm). From the spatial distribution, the deviation is between 0 and 1 mm in the upstream, while the value is larger (∼2 mm) in the downstream.
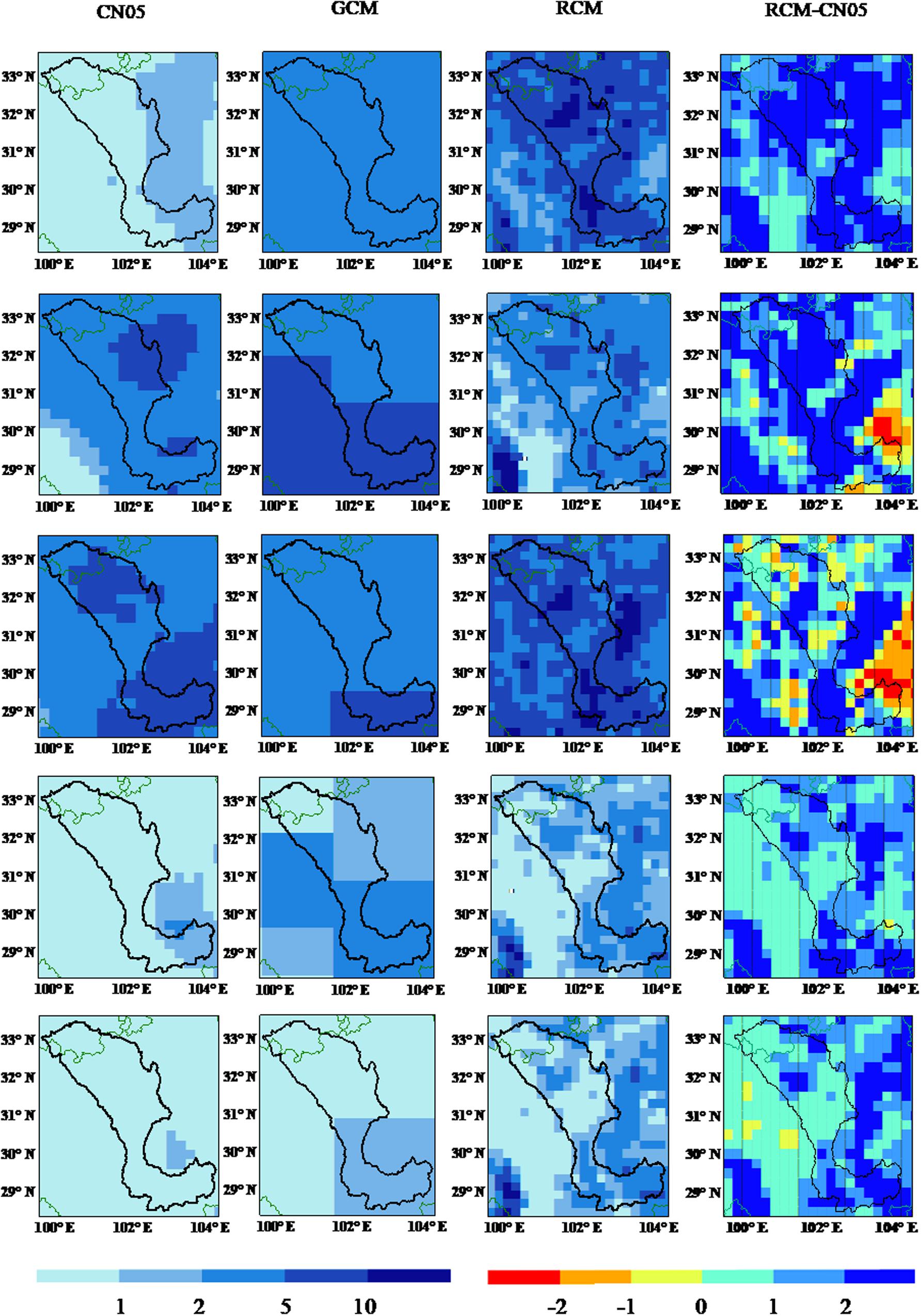
Figure 5. Simulation results and deviation of difference scale models in each period.
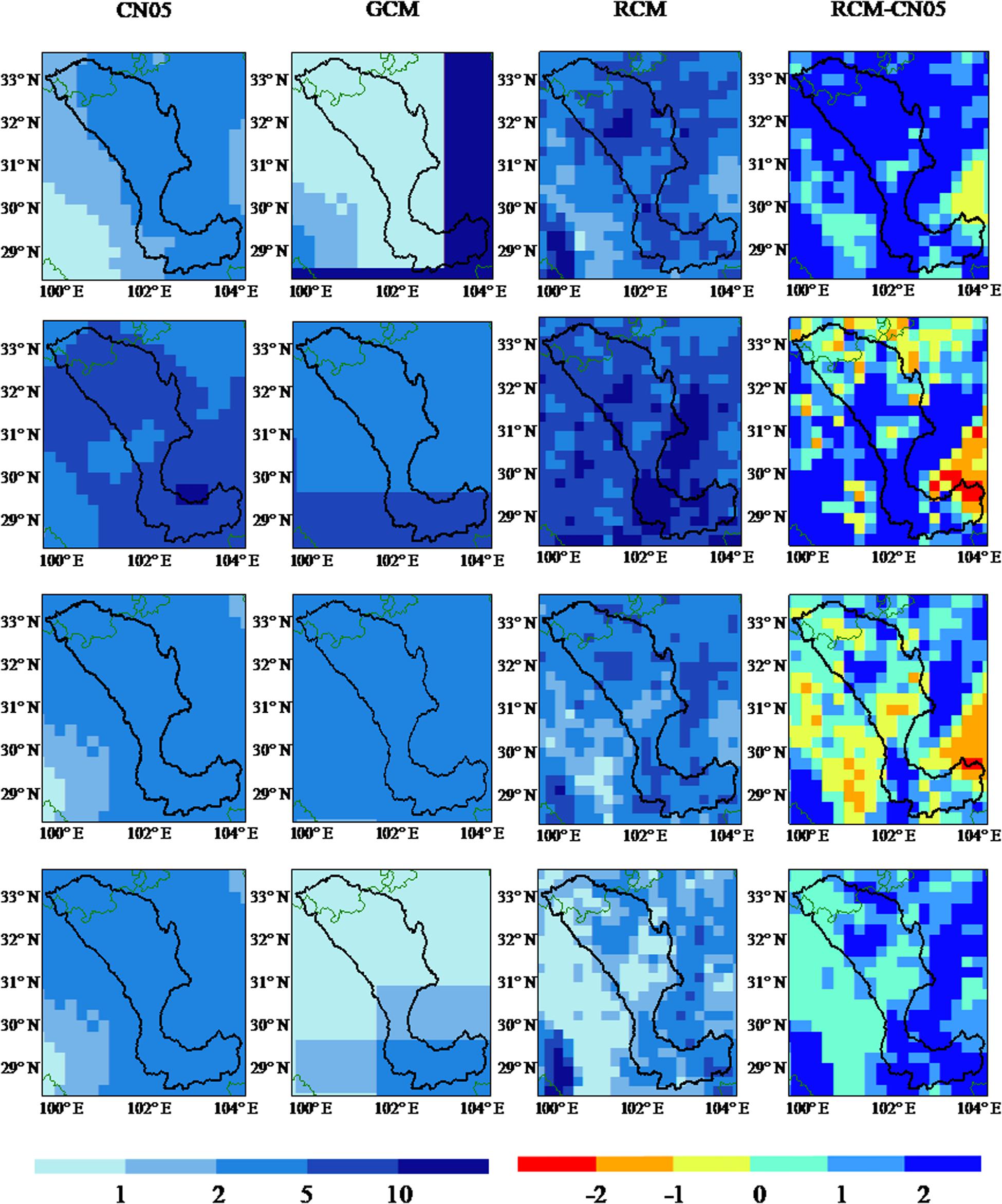
Figure 6. Simulation results and deviation of difference scale models in each season.
Figure 7 shows simulation results in annual cycle obtained from GCM and PRECIS. Compared to CN05, PRECIS can simulate the monthly precipitation in Dadu River Basin reasonably. For example, the highest precipitation occurs in June, while the precipitation in January is smaller. Meanwhile, PRECIS shows a great advantage in simulating the monthly mean precipitation relative to its driving GCM. Lots of overestimations in HadGEM2-ES (especially from May to October) are corrected by PRECIS in dynamic downscaling. Overall, the bias of RCM is kept between −0.34 and 3.67 mm, especially in August (∼−0.34 mm).
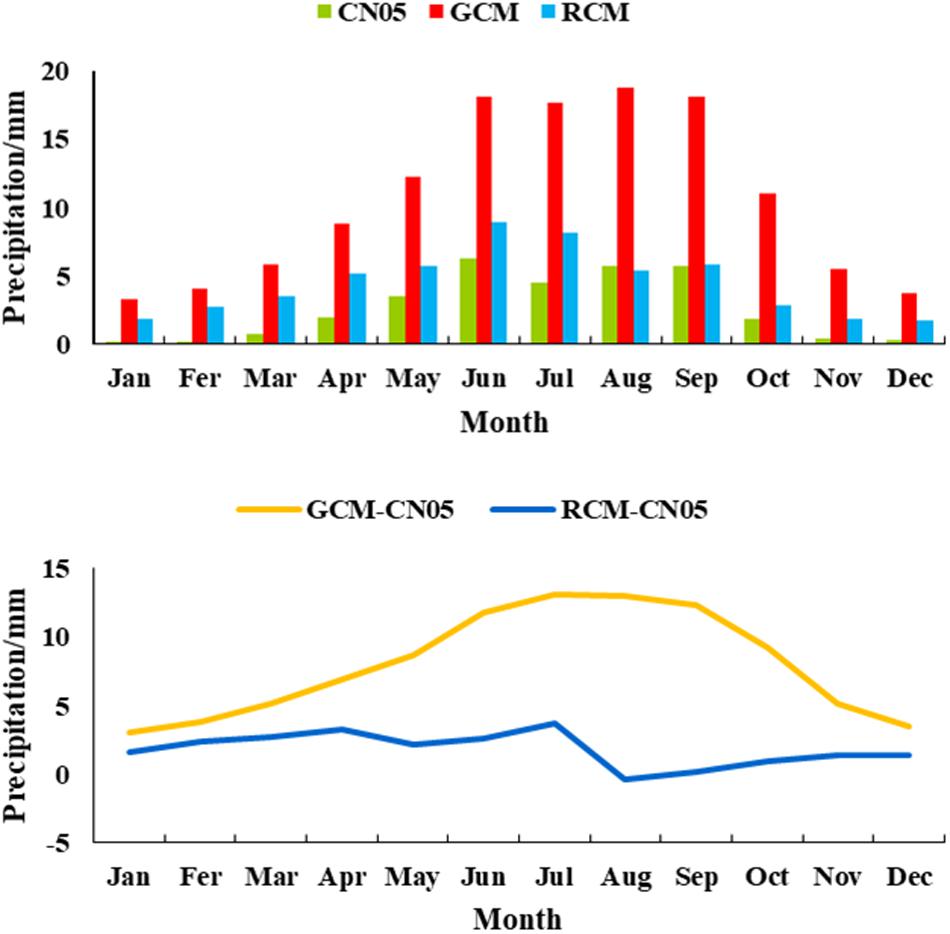
Figure 7. Monthly mean precipitation and deviation of different scale models and observation data.
Figure 8 is the comparison results between simulation and observation in precipitation data at different spatial scales. The higher in resolutions for climate model, the simulation trend and value of precipitation are closer to the observed data. Specifically, comparing to observation, the simulation results of GCM are overestimated obviously, especially in June or Neutral I, the error is even exceeding 20 mm/day. While the results downscaled by PRECIS have numerous improvements relative to its GCM. However, PRECIS still cannot solve effectively the accuracy in simulating station-scale precipitation. For example, the error is still high in June (about 10 mm) and in Neutral I (about 8 mm). On the other hand, the results from SCA statistical downscaling show the best performance than other simulations. The simulated precipitation is consistent with the observation well. The errors in GCM and PRECIS are reduced greatly, particularly in August and Dry II.
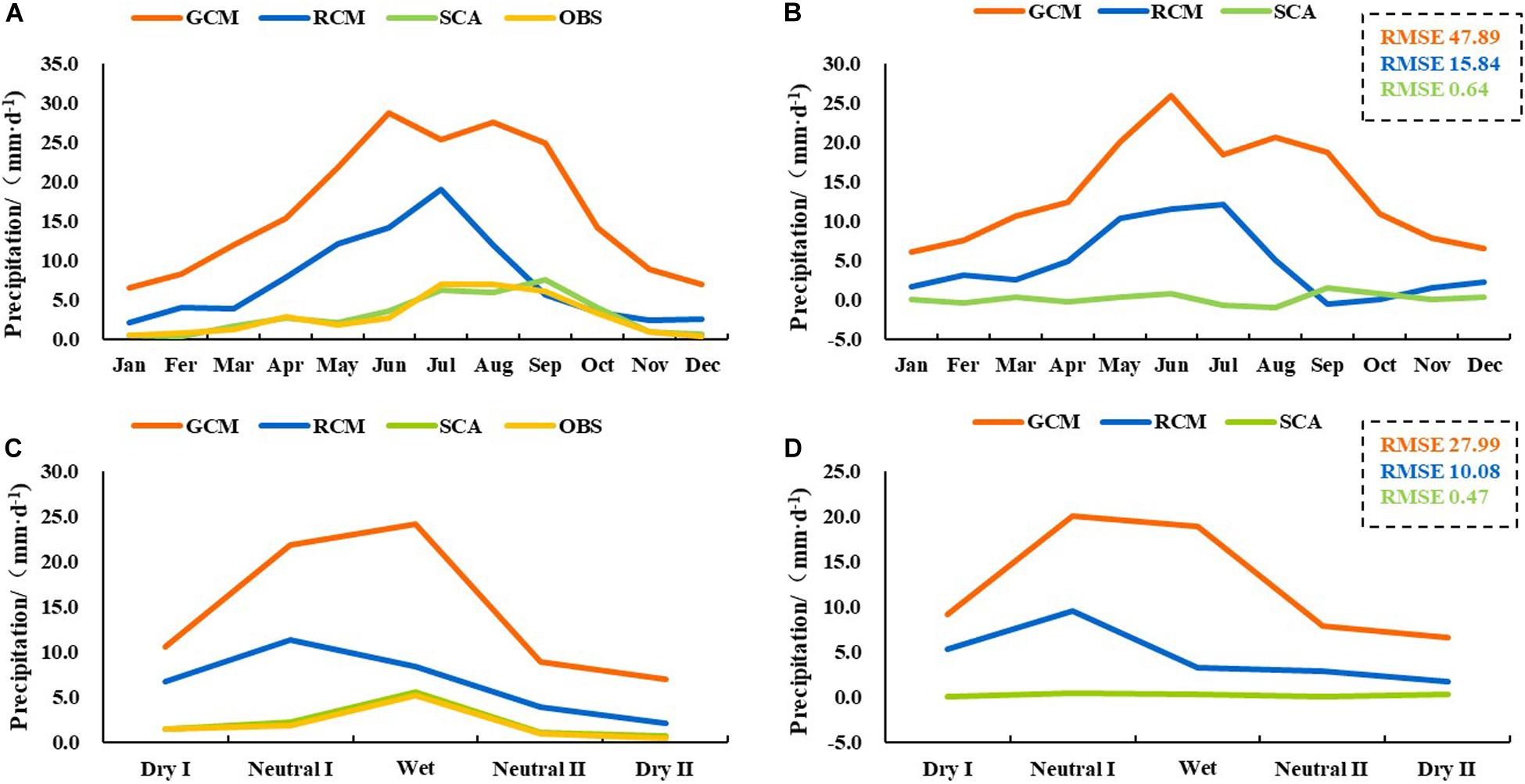
Figure 8. Comparison of precipitation simulation and observed data in different scale models: (A) monthly precipitation; (B) monthly precipitation error; (C) precipitation in period; (D) precipitation error in period.
In terms of the correlations between simulations and observation, SCA downscaling also show its advantage, with a correlation coefficient (about 0.97) and low RMSE value (about 0.16), meaning that there is a great fitting degree and lower biases between the results and observation ( Figure 9 ). Moreover, with the improvement of resolution, the decrease of RMSE is more pronounced. For example, the RMSE is about 68.9 mm/day in July for GCM and about 10.2mm/day for PRECIS, while the RMSE of SCA statistical downscaling is only about 0.5 mm/day. In addition, the RMSEs are different in periods and SCA shows a better performance (∼ 0.16 mm/day) in Dry II periods than that in other periods.
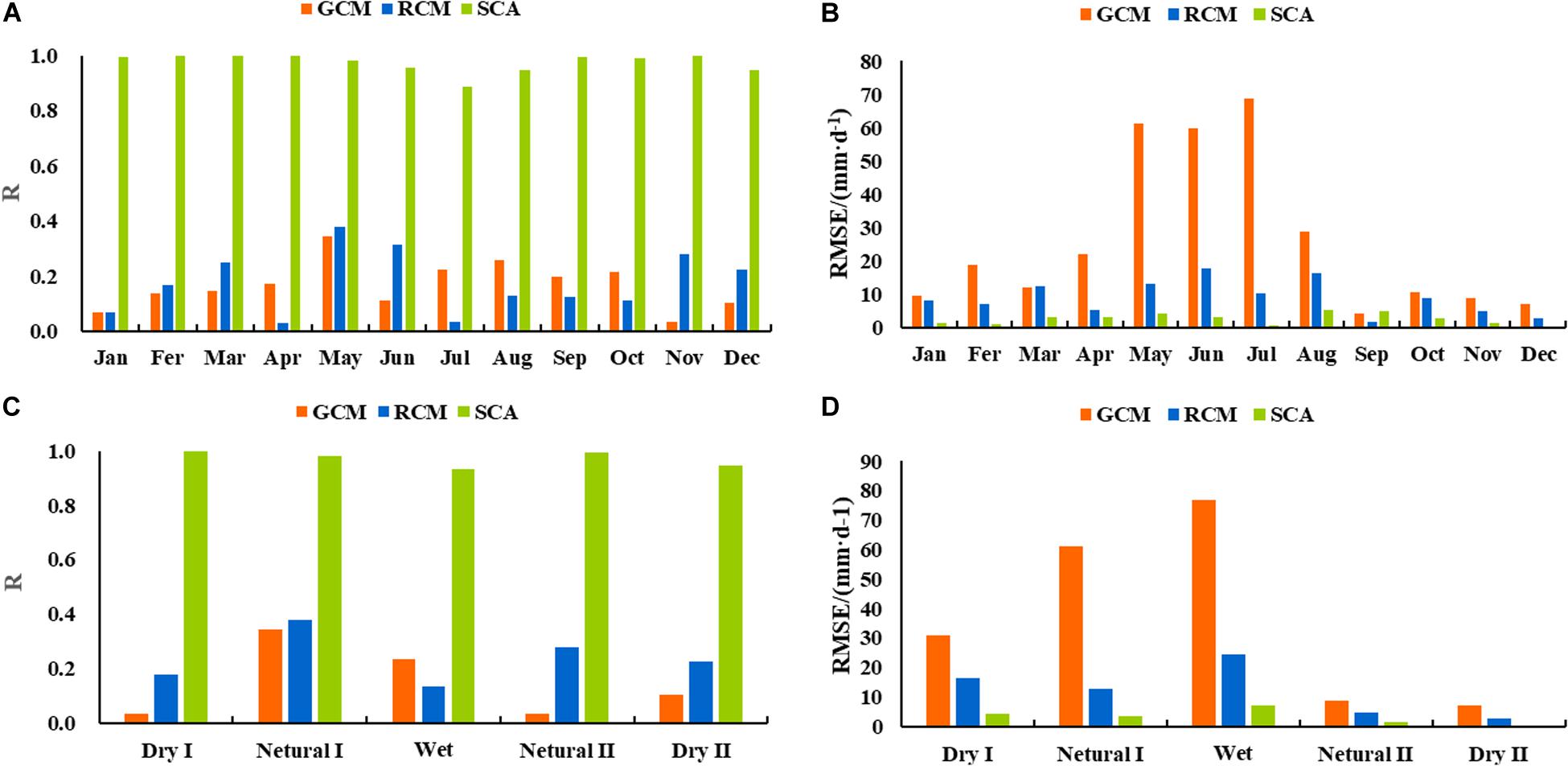
Figure 9. Verification of precipitation simulation and observed data in different scale models: (A) monthly correlation coefficient; (B) monthly RMSE; (C) correlation coefficient in period; (D) RMSE in period.
Overall, compared with coarser-resolution climate models, the simulation results of SCA statistical downscaling can reasonably reproduce the precipitation trend and value. Thus, these results can be used as the input of a subsequent power forecasting model to simulate the power generation of hydropower stations.
Then, through the HRMDP model, we compare the simulated power generation and actual power generation in different months and periods for Station A, which is shown in Figure 10 . Overall, the performance in correlation coefficients and RMSE values are quite satisfactory especially from May to July, which are 0.85 and 232 kWh respectively. The simulation results demonstrate a great performance in Wet period, and the correlation coefficients and RMSE values in different periods are 0.95 and 611 kWh, respectively.
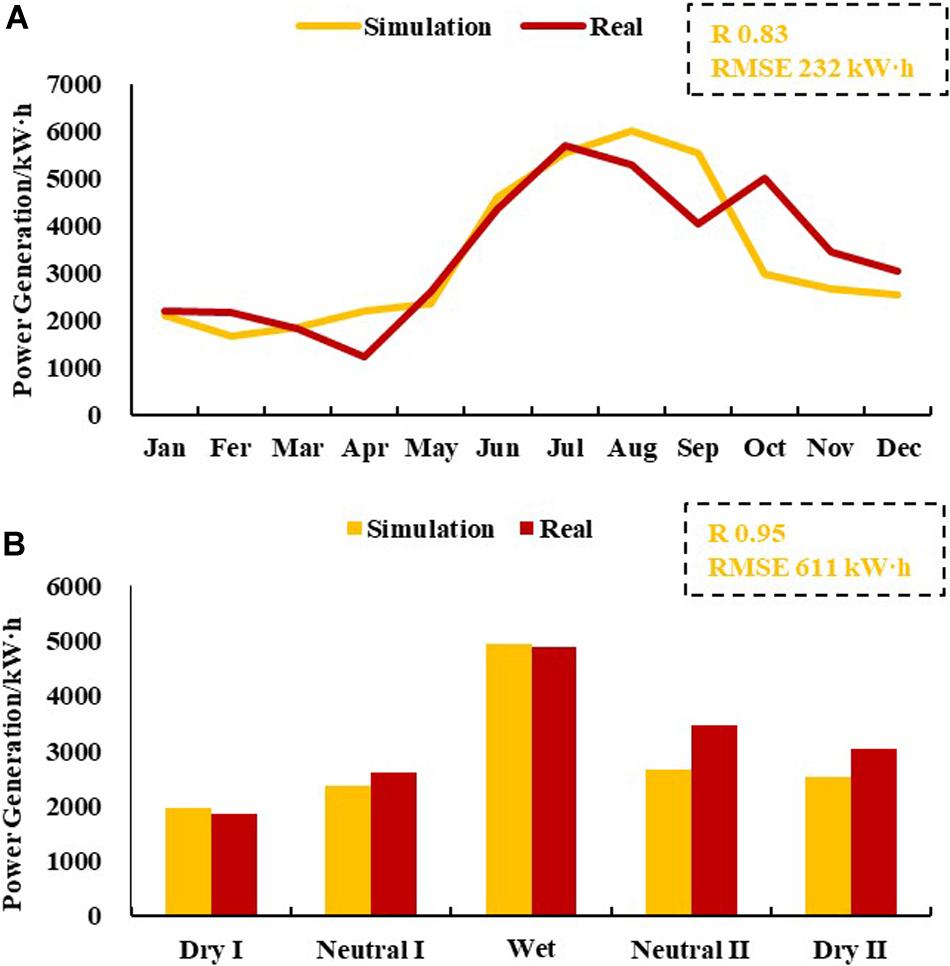
Figure 10. Simulation power generation compare with actual power generation: (A) monthly power generation; (B) power generation in period.
Future Forecasting
The projection in precipitation is the first step to forecast the future power generation through the HRMDP model. The future precipitation and percentage change through SCA downscaling in annual cycle and different periods are shown in Figure 11 . Compared with the historical period, the projected precipitation in annual cycle shows different change trends. Specifically, precipitation will increase by about 20–80% from May to July, while it will decrease in other months. For different periods, precipitation will increase in Neutral I and Wet, which are 79 and 4% respectively. The projected precipitation in other periods shows a decreasing trend, particularly in Neutral II (about 58%).
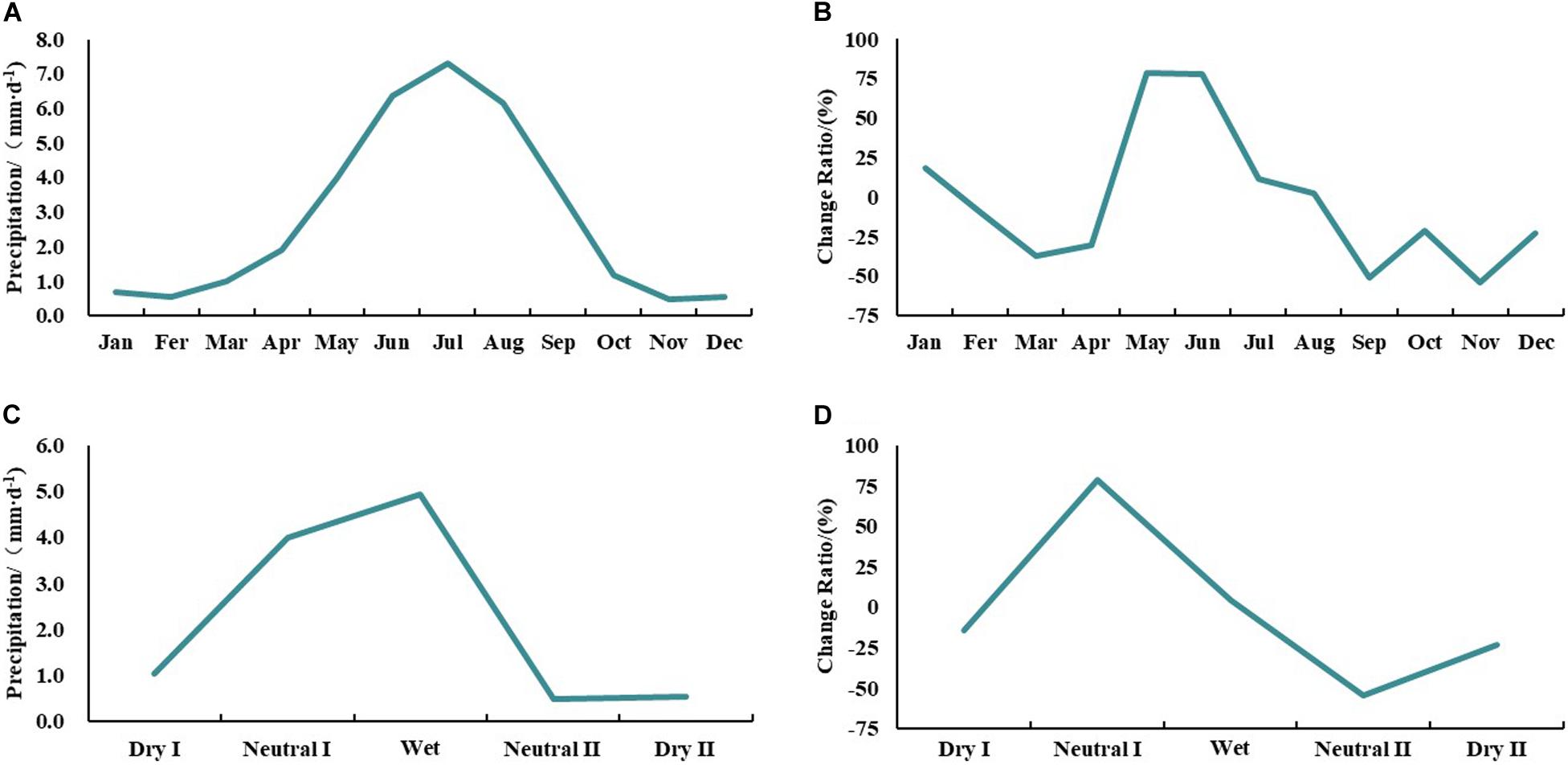
Figure 11. Future precipitation forecasting: (A) future monthly precipitation; (B) monthly precipitation change; (C) future precipitation in period; (D) precipitation change in period.
The changes in power generation resulting from the precipitation changes in station A in the future are shown in Figure 12 . Compared with the historical period, there is an ascending trend in power generation in station A from January to February and May to July in the future, especially in February with the most increase by about 25%. However, in the rest months, there is a decreasing trend in power generation in station A, for example, the decrease in September will be 36%. Similarly, the power generation will increase by about 20% in future Neutral I period, while there is no obvious change in other three periods relative to the historical period.
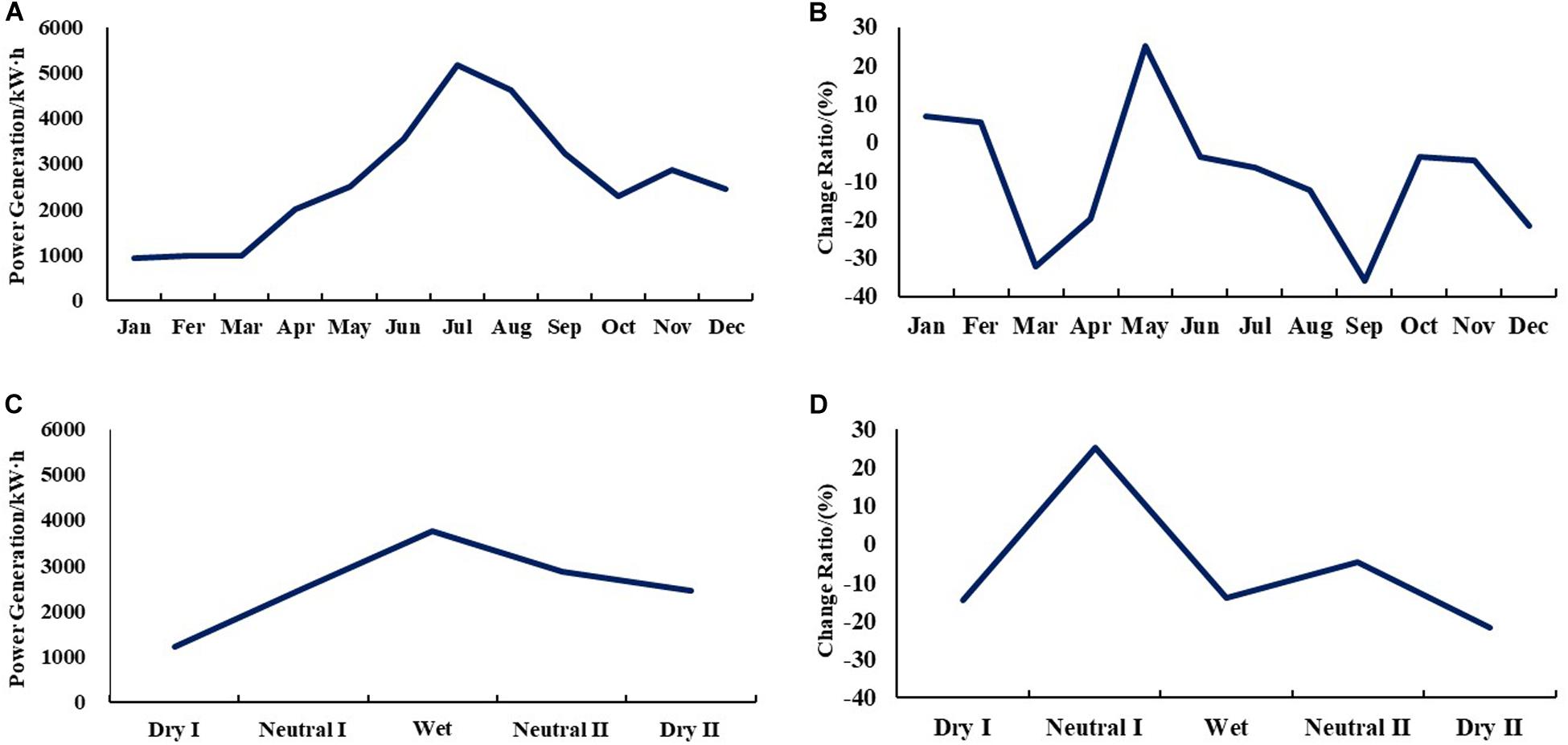
Figure 12. Future power generation change: (A) future monthly power generation; (B) monthly power generation change; (C) future power generation in period; (D) power generation change in period.
It is noted that there is more power generation in September in tradition, but the decrease in future precipitation will lead to a decrease in power generation in this month. On the other hand, though the power generation is less in May in the historical, the value will increase because of the increased precipitation in future. In addition, the peak of the generation will change from July to August, and the value will decrease about 8%. Meanwhile, the peak period of power generation will be shortened from June to August, because of the influence of precipitation.
Conclusion and Discussion
In this paper, based on the results from HadGEM2-ES and PRECIS climate models, the precipitation and power generation in power station A over Dadu River basin in China were simulated and projected using SCA statistical downscaling. The results from SCA downscaling show a great performance relative former climate models in simulating the precipitation at station scale. On the other hand, we used the observed meteorological and hydropower data in station A to establish a HRMDP. Meanwhile, we also validated the performance of HRMDP, and the results show that the HRMDP model can reflect the relationship between the precipitation and power generation, with the correlation coefficient of 0.95 and RMSE of 611 kWh.
In future, the precipitation of station A is projected an ascending trend from May to July while it will decrease in other months. For different periods, the precipitation will increase in Neutral I and Wet, but it will decrease in other periods. As the response from precipitation, the power generation will also show a similarly trend. There is an increasing trend in power generation in station A from January to February and May to July, while there is a decreasing trend in other months. For different periods, the power generation in Neutral I will increase by about 20% in future, while there is no obvious change in other three periods relative to the historical period.
Climate change has a great impact on precipitation, which affects hydropower generation. Therefore, it is of great significance to study the future precipitation change and power generation forecasting model of hydropower station to adapt climate change. As far as we know, there are many studies on future precipitation changes using climate models, but relatively few on hydropower generation forecasting model. For example, for precipitation, future climate change will lead to a general increase in precipitation over the most regions of China ( Lin and Zhou, 2015 ; Zhao et al., 2019 ). Future precipitation of Dadu river basin in our study also shows an increase trend, which is consistent with other similar basins ( Zhang J. et al., 2013 ; Zheng et al., 2017 ). In addition, although there are many researches on power generation forecasting methods, which focus on the runoff forecast, there are few studies on hydropower generation forecasting directly. For example, Cheng et al. (2013) used linear trend estimation method, Morlet wavelet transform and Kendall rank correlation method to forecast future runoff over the Dadu river basin, and shows that runoff will appear an increase trend. Our results show that hydropower generation has an increase trend in the future, which means the increase of precipitation will lead to increase of runoff, and further promote the increase of hydropower generation. The results can provide some decision support for future water resources management in Dadu river basin, especially for the planning and operation of hydropower stations.
Data Availability Statement
The datasets generated for this study are available on request to the corresponding author.
Author Contributions
LW, LJ, and GJ conceived and designed the research, collected the data, and performed the data analyses and manuscript preparation. LW, LJ, GJ, and BZ formulated the optimization model. LW, LJ, GJ, and FL wrote the manuscript. LW, GJ, and HB gave the comments and helped to revise the manuscript. All authors contributed to the article and approved the submitted version.
This research was supported by the National Key Research and Development Plan (2018YFE0196000), Natural Sciences Foundation (51190095), and the 111 Project (B14008).
Conflict of Interest
The authors declare that the research was conducted in the absence of any commercial or financial relationships that could be construed as a potential conflict of interest.
Ali, S. A., Aadhar, S., Shah, H. L., and Mishra, V. (2018). Projected increase in hydropower production in india under climate change. Sci. Rep. 8:12450.
Google Scholar
Arnette, A. N. (2017). Renewable energy and carbon capture and sequestration for a reduced carbon energy plan: An optimization model. Renew. Sustain. Energy Rev. 70, 254–265. doi: 10.1016/j.rser.2016.11.218
CrossRef Full Text | Google Scholar
Bellouin, N., Rae, J., Jones, A., Johnson, C., Haywood, J., and Boucher, O. (2011). Aerosol forcing in the Climate Model Intercomparison Project (CMIP5) simulations by HadGEM2-ES and the role of ammonium nitrate. J. Geophys. Res. 116, 2020610–2021029.
Berghuijs, W. R., Woods, R. A., and Hrachowitz, M. (2014). A precipitation shift from snow towards rain leads to a decrease in streamflow. Nat. Climate Change 4, 583–586. doi: 10.1038/nclimate2246
Chen, J., Brissette, F. P., and Leconte, R. (2011). Uncertainty of downscaling method in quantifying the impact of climate change on hydrology. J. Hydrol. 401, 190–202. doi: 10.1016/j.jhydrol.2011.02.020
Chen, X., and Zong, Y. (2000). The 1998 flood on the Yangtze, China. Nat. Hazard 22, 165–184.
Cheng, K., Zhou, D., Li, M., and Zhan, W. (2013). Characteristic analysis of precipitation and runoff in Daduhe River in Last 51 Years. Water Resour. Power 31:5-8+240.
Chilkoti, V., Bolisetti, T., and Balachandar, R. (2017). Climate change impact assessment on hydropower generation using multi-model climate ensemble. Renew. Energy 109, 510–517. doi: 10.1016/j.renene.2017.02.041
Chu, S., and Majumdar, A. (2012). Opportunities and challenges for a sustainable energy future. Nature 488, 294–303. doi: 10.1038/nature11475
PubMed Abstract | CrossRef Full Text | Google Scholar
CREO (2018). China Renewable Energy Outlook 2018. Available online at: http://boostre.cnrec.org.cn/wp-content/uploads/2018/11/China-Renewable-Energy-Outlook-2018-Folder_ENG.pdf (accessed December 23, 2018).
Duan, K., and Mei, Y. (2013). A comparison study of three statistical downscaling methods and their model-averaging ensemble for precipitation downscaling in China. Theor. Appl. Climatol. 116, 707–719. doi: 10.1007/s00704-013-1069-8
Guo, J., Huang, G., Wang, X., Li, Y., and Lin, Q. (2017a). Dynamically-downscaled projections of changes in temperature extremes over China. Climate Dyn. 50, 1045–1066. doi: 10.1007/s00382-017-3660-7
Guo, J., Huang, G., Wang, X., Li, Y., and Lin, Q. (2017b). Investigating future precipitation changes over China through a high-resolution regional climate model ensemble. Earth’s Future 5, 285–303. doi: 10.1002/2016ef000433
Guo, J., Huang, G., Wang, X., Li, Y., and Yang, L. (2018). Future changes in precipitation extremes over China projected by a regional climate model ensemble. Atmospher. Environ. 188, 142–156. doi: 10.1016/j.atmosenv.2018.06.026
Hui, P., Tang, J., Wang, S., Niu, X., Zong, P., and Dong, X. (2018). Climate change projections over China using regional climate models forced by two CMIP5 global models. Part II: projections of future climate. Int. J. Climatol. 38, e78–e94. doi: 10.1002/joc.5409
IHA (2018). iha_2018_hydropower_status_report_4.pdf. London: IHA.
IPCC (2013). IPCC fifth assessment report. Weather 68, 310–310.
Jiang, Z., Li, R., Li, A., and Ji, C. (2018). Runoff forecast uncertainty considered load adjustment model of cascade hydropower stations and its application. Energy 158, 693–708. doi: 10.1016/j.energy.2018.06.083
Jones, C. D., Hughes, J. K., Bellouin, N., Hardiman, S. C., Jones, G. S., Knight, J., et al. (2011). The HadGEM2-ES implementation of CMIP5 centennial simulations. Geosci. Model Dev. 4, 543–570. doi: 10.5194/gmd-4-543-2011
Kerkhoff, C., Künsch, H. R., and Schär, C. (2014). Assessment of Bias assumptions for climate models. J. Climate 27, 6799–6818. doi: 10.1175/jcli-d-13-00716.1
Li, Y., and Yan, X. (2017). Statistical downscaling of monthly mean temperature for Kazakhstan in Central Asia. Climate Res. 72, 101–110. doi: 10.3354/cr01456
Lin, R., and Zhou, T. (2015). Reproducibility and future projections of the precipitation structure in east asia in four chinese gcms that participated in the CMIP5 experiments. Chin. J. Atmosph. Sci. 39, 338–356.
Liu, X., Tang, Q., Voisin, N., and Cui, H. (2016). Projected impacts of climate change on hydropower potential in China. Hydrol. Earth Syst. Sci. 20, 3343–3359. doi: 10.5194/hess-20-3343-2016
Liu, Z., Xu, Z., Charles, S. P., Fu, G., and Liu, L. (2011). Evaluation of two statistical downscaling models for daily precipitation over an arid basin in China. International J. Climatol. 31, 2006–2020. doi: 10.1002/joc.2211
Panwar, N. L., Kaushik, S. C., and Kothari, S. (2011). Role of renewable energy sources in environmental protection: A review. Renew. Sustain. Energy Rev. 15, 1513–1524. doi: 10.1016/j.rser.2010.11.037
Perkins, S. E., Pitman, A. J., Holbrook, N. J., and McAneney, J. (2007). Evaluation of the AR4 Climate Models’ Simulated Daily Maximum Temperature, Minimum Temperature, and Precipitation over Australia Using Probability Density Functions. J. Climate 20, 4356–4376. doi: 10.1175/jcli4253.1
REN21 (2018). Renewable 2018.pdf. Paris: REN21.
Saini, R., Wang, G., Yu, M., and Kim, J. (2015). Comparison of RCM and GCM projections of boreal summer precipitation over Africa. J. Geophys. Res. 120, 3679–3699. doi: 10.1002/2014jd022599
Wang, X., Huang, G., Lin, Q., Nie, X., and Liu, J. (2015). High-resolution temperature and precipitation projections over Ontario. Canada: a coupled dynamical-statistical approach. Q. J. R. Meteorol. Soc. 141, 1137–1146. doi: 10.1002/qj.2421
Wasko, C., and Sharma, A. (2017). Global assessment of flood and storm extremes with increased temperatures. Sci. Rep. 7:7945.
Wood, A. W., Leung, L. R., Sridhar, V., and Lettenmaier, D. P. (2004). Hydrologic implications of dynamical and statistical approaches to downscaling climate model outputs. Clim. Change 62, 189–216. doi: 10.1023/b:clim.0000013685.99609.9e
Xu, Y., Gao, X., Shen, Y., Xu, C., Shi, Y., and Giorgi, F. (2009). A daily temperature dataset over China and its application in validating a RCM simulation. Adv. Atmosph. Sci. 26, 763–772. doi: 10.1007/s00376-009-9029-z
Yang, C., Wang, N., Wang, S., and Zhou, L. (2016). Performance comparison of three predictor selection methods for statistical downscaling of daily precipitation. Theor. Appl. Climatol. 131, 43–54. doi: 10.1007/s00704-016-1956-x
Yang, Y., Tang, G., Lei, X., Hong, Y., and Yang, N. (2017). Can satellite precipitation products estimate probable maximum precipitation: a comparative investigation with gauge data in the dadu river Basin. Remote Sens. 10:41. doi: 10.3390/rs10010041
Zhang, D.-L., Lin, Y., Zhao, P., Yu, X., Wang, S., Kang, H., et al. (2013). The Beijing extreme rainfall of 21 July 2012: “Right results” but for wrong reasons. Geophys. Res. Lett. 40, 1426–1431. doi: 10.1002/grl.50304
Zhang, J., Luo, L., Han, X., and Zhang, C. (2013). Simulation and Prediction of annual Precipitation in the Yangtze River Basin by CMIP5 model. Guizhou Meteorological Society. Guizhou Meteorological Society: Guizhou Association for Science and Technology, 2013:6.
Zhang, Z., and Xu, J. (2014). Applying rough random MODM model to resource-constrained project scheduling problem: A case study of Pubugou Hydropower Project in China. KSCE J. Civil Eng. 18, 1279–1291. doi: 10.1007/s12205-014-0426-1
Zhao, Y., Xiao, D., and Pai, H. (2019). Projection and application for future climate in china by CMIP5 climate model. Meteorol. Sci. Technol. 47, 608–621. doi: 10.1002/joc.5409
Zheng, W., Cheng, X., Yang, X., and Wang, Y. (2017). Trend analysis of precipitation and temperature over the upper changjiang river basin by statistical-downscaled cmip 5 climate projections. China Rural Water Hydropower (09):43-47+51.
Keywords : hydropower, precipitation, regional climate model, stepwise clustering analysis, downscaling
Citation: Wei L, Jiheng L, Junhong G, Zhe B, Lingbo F and Baodeng H (2020) The Effect of Precipitation on Hydropower Generation Capacity: A Perspective of Climate Change. Front. Earth Sci. 8:268. doi: 10.3389/feart.2020.00268
Received: 28 October 2019; Accepted: 15 June 2020; Published: 08 September 2020.
Reviewed by:
Copyright © 2020 Wei, Jiheng, Junhong, Zhe, Lingbo and Baodeng. This is an open-access article distributed under the terms of the Creative Commons Attribution License (CC BY) . The use, distribution or reproduction in other forums is permitted, provided the original author(s) and the copyright owner(s) are credited and that the original publication in this journal is cited, in accordance with accepted academic practice. No use, distribution or reproduction is permitted which does not comply with these terms.
*Correspondence: Li Jiheng, [email protected] ; Guo Junhong, [email protected]
This article is part of the Research Topic
Future Climate Scenarios: Regional Climate Modelling and Data Analysis
A Hydroelectric Power Plant Brief: Classification and Application of Artificial Intelligence
Ieee account.
- Change Username/Password
- Update Address
Purchase Details
- Payment Options
- Order History
- View Purchased Documents
Profile Information
- Communications Preferences
- Profession and Education
- Technical Interests
- US & Canada: +1 800 678 4333
- Worldwide: +1 732 981 0060
- Contact & Support
- About IEEE Xplore
- Accessibility
- Terms of Use
- Nondiscrimination Policy
- Privacy & Opting Out of Cookies
A not-for-profit organization, IEEE is the world's largest technical professional organization dedicated to advancing technology for the benefit of humanity. © Copyright 2024 IEEE - All rights reserved. Use of this web site signifies your agreement to the terms and conditions.
Advertisement
A study on solutions and problems of hydroelectric power plants in the operation
- Original Article
- Published: 29 May 2022
- Volume 8 , article number 90 , ( 2022 )
Cite this article
- Cengiz Koç ORCID: orcid.org/0000-0001-7310-073X 1
420 Accesses
3 Citations
Explore all metrics
Hydroelectricity, which is defined as green energy, is a type of renewable energy. Despite the advantages of hydroelectric power plants, there are also negative effects caused by them. While power plants do provide advantages such as flood reduction, disaster management, mitigation of the effects of global warming and climate change; they can also lead to some unwanted environmental effects at the local and regional levels. In this study, the generation capacities of hydroelectric power plants that were built and commissioned by a Public Institution or by the Private Sector in the Büyük Menderes and Western Mediterranean basins were evaluated, for the years of 2010–2019. In the years examined, the project energy production value was compared with the maximum and minimum energy production levels of the power plants. The average maximum and average minimum production rates, which were calculated by proportioning the maximum and minimum energy production values to the project production value, was 93.33% and 56.04% for the West Mediterranean basin, respectively; and it was 92.97% and 43.09% for the Büyük Menderes basin. The average production rate for the Western Mediterranean basin was 69.8%, and for the Büyük Menderes basin it was 68%. It has been shown that there were significant differences between the annual energy production of the power plants and the amount of production realized due to the changes in the water resources of the studied basins, as well as due to insufficient data and careless planning. In addition, the production and environmental problems encountered during the operation phase have been revealed and suggestions have been recommended for the resolution of these problems in this study.
This is a preview of subscription content, log in via an institution to check access.
Access this article
Price includes VAT (Russian Federation)
Instant access to the full article PDF.
Rent this article via DeepDyve
Institutional subscriptions
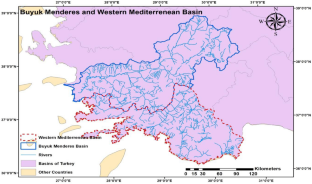
Similar content being viewed by others
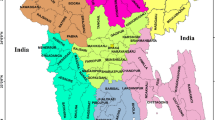
Hydropower potentials in Bangladesh in context of current exploitation of energy sources: a comprehensive review
S. K. Sen, A. H. Al Nafi Khan, … U. Sumaiya
Hydropower Potential in India: A Review
Hydropower Plants
Abbasi T, Abbasi SA (2011a) Small hydro and the environmental implications of its extensive utilization. Renew Sust Energ Rev 15(4):2134–2143
Article Google Scholar
Abbasi T, Abbasi SA (2011b) Small hydro and the environmental implications of its extensive utilization. Renew Sustain Energy Rev 15:2134–2143
Anderson D, Moggridge H, Warren P, Shucksmith J (2015) The impacts of ‘run-of-river’ hydropower on the physical and ecological condition of rivers. Water Environ J 29:268–276
Avcı I (2008) Goals, expectations and realities in the practice. New global perspectives in the assessment and management of hydroelectric potential and water, a strategic resource in Turkey. Union of Chambers of Turkish Engineers and Architects, Journal of Measure, January 42–42. (in Turkish)
Bayazıt Y, Bakış R, Koç C (2017) An investigation of small scale hydropower plants using the geographic information system. Renew Sustain Energy Rev 67:289–294
Bayazıt Y, Bakış R, Koç C (2021) A study on transformation of multi-purpose dams into pumped storage hydroelectric power plants by using GIS model. Int J Green Energy 18(3):308–318
Bunn SE, Arthington AH (2002) Basic principles and ecological consequences of altered flow regimes for aquatic biodiversity. Environ Manag 30:492–507
Cejka T, Beracko P, Matecny I (2019) The impact of the Gabcikovo hydroelectric power barrier on the Danube floodplain environment-the results of long-term monitoring of land snail fauna. Environ Monit Assess 192:30
Couto TBA, Olden JD (2018) Global proliferation of small hydropower plants—science and policy. Front Ecol Environ 16:91–100
Dogmus ÖC, Nielsen JO (2020) The on-paper hydropower boom: a case study of corruption in the hydropower sector in Bosnia and Herzegovina. Ecol Econ 172:106630
DSI (2019) Büyük Menderes ve Batı Akdeniz Havzalarında İşletilen Hidroelektrik Santral Kayıtları, Tarım ve Orman Bakanlığı, DSİ Genel Müdürlüğü, DSİ 21. Bölge Müdürlüğü, İşletme ve Bakım Şube Müdürlüğü, Aydın
DSI (2020) http://www.dsi.gov.tr/english/service/enerjie.htm . Accessed 20 Apr 2020
EC (European Commission) (2000) Directive 2000/60/EC of the European Parliament and of the Council of 23 October 2000 establishing a framework for Community action in the field of water policy. Off J Eur Communities L 327:1–73
Google Scholar
EC (European Commission) (2018) Commission E. Guidance document on the requirements for hydropower in relation to EU nature legislation. In: Environment, editor. Brussels, Belgium, p 1–83
Erlewein A (2013) Disappearing rivers—the limits of environmental assessment for hydropower in India. Environ Impact Assess Rev 43:135–143
ESHA (2010) Hydropower and environment, technical and operational procedures to better integrate small hydropower plants in the environment. http://www.esha.be . Accessed Oct 2010
EÜAŞ (2008) Özel Sektörün Hidrolik Santral Yapma A¸samaları. Elektrik Üretim Anonim Sirketi. http://www.euas.gov.tr/ . Accessed 12 May 2020
EUROSTAT (2019) Energy statistics introduced. https://ec.europa.eu/eurostat/statistics-explained/index.php?title=Energy_statistics_introduced#Introduction . Accessed 1 May 2020
ICOLD (2000) Open letter to WCD Chair, 30 November 2000. International Commission on Large Dams
IHA (International Hydropower Association) (2019) Hydropower downstream flow regimes. International Hydropower Association, London, p 73
Kallis G, Butler D (2001) The EU water framework directive: measures and implications. Water Policy 3:125–142
Kelly S (2019) Megawatts mask impacts: small hydropower and knowledge politics in the Puelwillimapu, Southern Chile. Energy Res Soc Sci 54:224–235
Kelly-Richards S, Silber-Coats N, Crootof A, Tecklin D, Bauer C (2017) Governing the transition to renewable energy: a review of impacts and policy issues in the small hydropower boom. Energy Policy 101:251–264
Kentel E, Alp E (2013) Hydropower in Turkey: economical, social and environmental aspects and legal challenges. Environ Sci Policy 31:34–43
Koç C (2012) Problems and solutions related to hydroelectric power plants constructed on the Buyuk Menderes and the West Mediterranean basin. Energy Sources Part A Recov Util Environ Effects 34(15):1416–1425
Koç C (2014) A study on the development of hydropower potential in Turkey. Renew Sustain Energy Rev 39:498–508
Koç C (2017) A study on importance and role of irrigation and hydropower plant operation in integrated river basin management. Comput Water Energy Environ Eng 6:1–10
Koç C (2018) A study on operation problems of hydropower plants integrated with irrigation schemes operated in Turkey. Int J Green Energy 15(2):129–135
Koç C (2020a) A study on environmental and social impacts of mini hydro power plants. Eu J Sci Technol 20:35–41
Koç C (2020b) A study on operation of hydroelectric power plants constructed on irrigation canal. Eur J Sci Technol 19:138–144
Koç C, Kosif K, Kızıltepe S, Özdemir K (2010) Büyük Menderes ve Batı Akdeniz Havzalarında İşletmede olan Taşkın Tesislerine Yapılan Müdahaleler Üzerine Bir Çalışma. II. Ulusal Taşkın Sempozyumu 22–24 Mart, Afyonkarahisar, 71–79
Koralay N, Kara O, Kezik U (2018) Effects of run-of-the-river hydropower plants on the surface water quality in the Solakli stream watershed, Northeastern Turkey. Water Environ J 32:412–421
Laguna M, Upadhyay D, Taylor S (2006) Flowing to the East: Small Hydro in developing countries, Renewable Energy World, pp 126–131
Lange K, Meier P, Trautwein C, Schmid M, Robinson CT, Weber C et al (2018) Basin-scale effects of small hydropower on biodiversity dynamics. Front Ecol Environ 16:397–404
Larinier M (2008) Fish passage experience at small-scale hydro-electric power plants in France. Hydrobiologia 609:97–108
Mbaka JG, Wanjiru M (2015) A global review of the downstream effects of small impoundments on stream habitat conditions and macroinvertebrates. Environ Rev 23:257–262
McManamay RA, Samu N, Kao SC, Bevelhimer MS, Hetrick SC (2015) A multi-scale spatial approach to address environmental effects of small hydropower development. Environ Manag 55:217–243
Nautiyal H, Goel V (2020) Sustainability assessment of hydropower projects. J Clean Prod 265:121661
Pascale G, Connors BM, Palen WJ (2017) Run-of-River hydropower and salmonids: potential effects and perspective on future research. Can J Fish Aquat Sci 74:1135–1149
Poff NL, Zimmerman JKH (2010) Ecological responses to altered flow regimes: a literature review to inform the science and management of environmental flows. Freshw Biol 55:194–205
Popescu VD, Munshaw RG, Shackelford N, Montesino Pouzols F, Dubman E, Gibeau P et al (2020) Quantifying biodiversity trade-offs in the face of widespread renewable and unconventional energy development. Sci Rep 10:7603
Premalatha M, Tabassum A, Abbasi T, Abbasi SA (2014) A critical view on the eco-friendliness of small hydroelectric installations. Sci Total Environ 481:638–643
Ptak T (2019) Towards an ethnography of small hydropower in China: rural electrification, socioeconomic development and furtive hydroscapes. Energy Res Soc Sci 48:116–130
Rex W, Foster V, Lyon K, Bucknall J, Liden R (2014) Supporting hydropower: an overview of the World Bank Group’s engagement. World Bank Group, Washington, DC
SPD (2019). Hidroenerji Raporu Yekdem’e Kayıtlı HES’lerin Üretim Verimliliği, Su Politikaları Derneği, Hidropolitik Akademi, Rapor No: 2019-2, Kavaklıdere-Ankara, s.28
Valero E (2012) Characterization of the water quality status on a stretch of River Lérez around a small hydroelectric power station. Water 4:815–834
Yuksel I (2010) As a renewable energy hydropower for sustainable development in Turkey. Renew Sustain Energy Rev 14:3213–3219
Zarfl C, Lumsdon AE, Berlekamp J, Tydecks L, Tockner K (2014) A global boom in hydropower dam construction. Aquat Sci 77:161–170
Download references
Acknowledgements
In this study, data on the hydroelectric power plants operated and constructed in the Büyük Menderes and Western Mediterranean basins were obtained from the General Directorate of State Hydraulic Works. Therefore, I would like to thank the relevant institution for its contribution and assistance to this study.
Author information
Authors and affiliations.
Department of City and Regional Planning, Muğla Sıtkı Koçman University, Muğla, Turkey
You can also search for this author in PubMed Google Scholar
Corresponding author
Correspondence to Cengiz Koç .
Additional information
Publisher's note.
Springer Nature remains neutral with regard to jurisdictional claims in published maps and institutional affiliations.
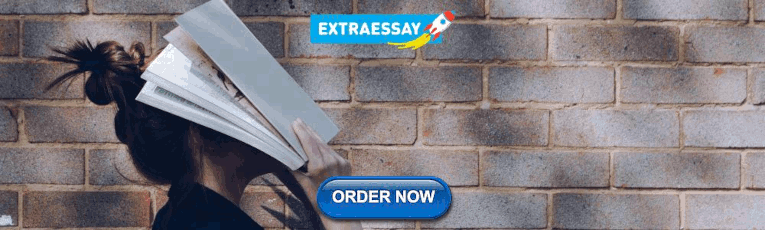
Rights and permissions
Reprints and permissions
About this article
Koç, C. A study on solutions and problems of hydroelectric power plants in the operation. Sustain. Water Resour. Manag. 8 , 90 (2022). https://doi.org/10.1007/s40899-022-00677-2
Download citation
Received : 30 July 2021
Accepted : 10 May 2022
Published : 29 May 2022
DOI : https://doi.org/10.1007/s40899-022-00677-2
Share this article
Anyone you share the following link with will be able to read this content:
Sorry, a shareable link is not currently available for this article.
Provided by the Springer Nature SharedIt content-sharing initiative
- Büyük Menderes
- Energy production rate
- Hydroelectric power plant
- Operational and environmental problems
- Western Mediterranean
- Find a journal
- Publish with us
- Track your research
- Original research article
- Open access
- Published: 01 March 2018
Conditions for economic competitiveness of pumped storage hydroelectric power plants in Egypt
- Diaa Abdellatif 1 ,
- Rameen AbdelHady 2 ,
- Ahmed M. Ibrahim 3 &
- Essam Abu El-Zahab 3
Renewables: Wind, Water, and Solar volume 5 , Article number: 2 ( 2018 ) Cite this article
15k Accesses
17 Citations
1 Altmetric
Metrics details
Pumped storage hydroelectric power plants are one of the most applicable energy storage technologies on large-scale capacity generation due to many technical considerations such as their maturity, frequency control and higher ramp rates, thus maintaining following loads in case of high penetration of renewables in the electrical grid. Economic viability of PSHPPs is still questionable when compared with other electricity generation technologies. In this manuscript, the key factors that affect the viability of constructing PSHPP in Attaqa Mountain, Egypt, are defined. An economic comparison between PSHPPs and simple cycle gas turbine power plants has been carried out to identify the conditions at which PSHPPs will have competitiveness as an on-peak solution, over traditional SCGT power plants. This assessment is based on calculating levelized cost of electricity taking into account different scenarios for PSHPP pumping cost and capital cost, and different scenarios for SCGT fuel prices. The results showed the key factors of the best case at which PSHPPs have the highest economic competitiveness over SCGT power plants when fuel prices are non-subsidized. This is only valid provided that the PSHPP’s capital cost should not exceed 4180 $/kW at zero pumping cost.
Introduction
Energy strategic planning is one of the world’s main concerns to cover the fast-growing energy demand taking into account the wise use of available energy resources to achieve the optimum electricity generation mix. One of the main difficulties of energy planning for any country is selecting the most compatible alternative to fulfill its requirements, as the selected alternative will have a long-term impact on its economy and the utilization of the available resources.
The on-peak electricity demand is a challenge for grid operators because it requires special generating power plants that can directly follow the increase in demand and maintain its balance with the supply. The electricity load duration curve of Egypt is not flat and consists of two major periods through the whole day: The first period is in day time from 1 am to 7 pm (off peak) during which the demand can be easily covered by base load generation power plants such as combined cycle, steam and simple cycle power plants, ordered, respectively, according to better economic dispatch and efficiencies and the second period is at night starting from 7 pm to 12 am (on-peak), as shown in Fig. 1 (EEHC 2015 ), at which electricity demand requires high ramp rate generation solutions (balancing the supply with the demand fluctuations over short times) that can quickly respond to the increase in that demand.
Egypt load curve 2014/2015
Typically, reservoir hydropower plants (HPPs) and PSHPPs supply primary control power. However, it is not the case for the Egyptian National Grid. In Egypt, the daily on-peak periods are met with peaking units, especially simple cycle gas turbine (SCGT) (specific), or using electrical interconnection between neighboring countries because they can quickly start up and shut down to meet brief peaks in demand. These solutions for on-peak periods are typically the most expensive to operate when considering peaking units and have low reliability when considering interconnection because it is dependent on other countries’ technical and political situations. Peaking units also serve as spinning reserve and as “quick start” units which are able to go from shutdown to full load in minutes. A peaking unit typically operates for only a few hundred hours a year. They run only as needed to meet the highest loads (Kaplan 2008 ).
Another solution that can cover on-peak demand through the day is electrical energy storage (EES) technologies whose operation is based on storing electrical energy when there is an excess generation then releasing this energy when needed, so this released electricity can promptly follow the electricity demand.
Many energy storage technologies are technically applicable, but they still have challenging issues related to the scale of their use, their capital costs and the installing site selection. Another challenge of energy storage technologies is the loss between charging and discharging energy that requires a specific technique of pricing and new energy market rules, thus increasing their economic viability.
This energy storage technology can provide two main services: supplying electricity during on-peak or high ramp demand rates and putting off some capacity expansions in the electrical grid.
EES technologies can be classified into different categories [Fig. 2 (OWOE 2014 )] according to their suitable storage durations, response times, functions or the form of energy they store.
Classification of EES technologies by the form of stored energy
One of the EES technologies is pumped hydro storage. In 2011, the International Hydro Power Association (IHA) estimated that pumped hydro storage capacity to be between 120 and 150 GW (IRENA 2012 ) with a central estimate of 136 GW. In 2014, the total installed capacity of pumped storage hydroelectric power plants (PSHPPs) around the world reached 140 GW, which is very large compared to other EES technologies (976 MW) (IEA 2014 ). Pumped storage represents about 2.2% of all generation capacity in the USA, 18% in Japan and 19% in Austria (IRENA 2012 ). According to energy storage exchange Web site statistics (Sandia National Laboratories 2016 ) in 2016, there are about 7 projects under construction until 2018 with a total capacity of 5.95 GW including 4 projects in Africa with total capacities of 2.05 GW in both Morocco and South Africa. There are many studies that have been done on different sites to construct a PSHPP in Egypt. The most viable site was found to be Attaqa Mountain (Fig. 3 ), based on the techno-economic study which has been done by a Swedish Consultant (SWECO 1997 ).
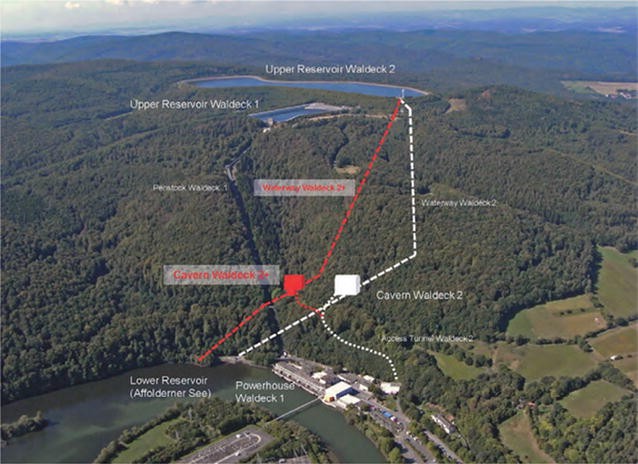
PSHPP of Attaqa Mountain, Egypt
According to this study, the site can involve six reversible turbines (overall flow rate 318 m 3 /s) with a total power capacity of 2100 MW with an upper reservoir, of 9 mcm volume capacity, at a height of 850 m. The horizontal distance between the upper and the lower reservoir is expected to be 1450 m; hence, the effective head is 600 m.
There are six vertical steel lined penstocks (with inner diameter of 3.6 m) for the flow of water up and down between the reservoirs, linked two by two to horizontal circular concrete lined tunnels with larger diameters. The access to the underground power plant is by a long tunnel, while there are three horizontal lower tunnels between the lower reservoir and the turbines. There will be a machinery hall (180 m long and 16 m wide) and a specific area (175 m long and 15 m wide) to include the power transformers (SWECO 1997 ).
The basic concept of PSHPPs involves pumping water from a lower reservoir for storage into an upper reservoir, then generating electricity by using the same water flowing from the upper reservoir through turbine generators back into the lower reservoir as shown in Fig. 4 (OWOE 2014 ). The cycle is repeated on daily or weekly basis.
PSHPP schematic diagram
This process can be operated using a reversible turbine to pump up water. The turbine is connected to a motor for generating electricity. The efficiency of PSHPPs to make full water cycle (cycle efficiency) ranges typically between 70 and 85% (Barbour et al. 2016 ). Therefore, the required energy to pump up water is higher than the generated energy from PSHPP by 15–30% due to losses, which is what the debate of PSHPPs is about. This can be solved by different tariff mechanisms (e.g., double tariff structure). Double tariff is the two times of use tariff (ToU) and it has two fixed values over the day based on times of configuration.
It is also possible to get benefit of excess electricity during lower-demand periods to charge water into upper reservoirs then this water can be discharged down to start up a turbine to generate electricity during the high-demand periods. The economic benefit of PSHPPs comes from pumping with low-cost off-peak and generating on-peak to displace high-cost energy, to reduce total generation cost by time shifting (IEC 2011 ). Moreover, PSHPP plays an important role in providing power factor correction and voltage regulation in the generating or pumping mode and they can also operate unloaded as synchronous condensers, thus enhancing the electrical power system stability.
The first designs of PSHPPs in the 1890s used separate pump motors and turbine generators. Since the 1950s, a single reversible pump turbine has become the dominant design for PSHPPs (Baxter 2006 ).
PSHPP has some advantages, when compared with SCGT power plants to cover the on-peak electricity demand; one of these advantages is that PSHPPs do not have the capacity reduction experienced by combustion turbines due to high ambient temperature, and at the same time, it has a higher reliability than combustion turbines. Other benefit of PSHPPs is their lifetimes because hydropower plants usually have very long lifetimes (in the range of 30–80 years) and their components can be sustained in operation (IRENA 2012 ).
Energy storage also has an important role in the efficient utilization of electricity from renewable energy resources. Many renewable energy resources, such as wind, solar, HPPs and tidal energy, are variable and inconstant and so are unable to supply electrical power continuously. Integrating some energy storage with renewable resources improves the expected generated energy from renewables, removes their uncertainties and increases the value of the electricity generated (Breeze 2014 ).
Although PSHPP and SCGT are technically viable to operate as on-peak generation power plants, the economic drawbacks of PSHPP due to its high capital cost and its cycle efficiency limit its feasibility. In this manuscript, a comparison of both PSHPP and SCGT in Egypt is presented. This comparison is based on a standard levelized cost of energy (LCOE) approach that enables decision makers to compare between different technologies of diverse lifetimes, capacities, heat rates and other specifications, to choose the most economic technology in $/kWh. LCOE is the summation of all the costs that are borne through a generating technology lifetime divided by the energy generated from that technology and is expressed in $/kWh.
The time value of money concept is also taken into consideration in calculating LCOE represented in the discount rate ( r ) which mathematically refers to the interest rate used to calculate the present value of future cash flow. LCOE of PSHPP and SCGT is calculated based on financial modeling, using Microsoft Excel spreadsheets, taking into consideration the different technical and economic aspects of each technology such as heat rate, lifetime, capacity factor, fixed and variable O&M and capital costs. An analysis of different scenarios is also performed to define the conditions at which PSHPP has competitiveness over SCGT power plants. These cases include scenarios for PSHPP pumping cost and scenarios for SCGT fuel costs which have a significant impact on the LCOE of both power plants; also, different values of r are considered. This is a simplified approach, as PSHPPs have many additional features of their operation in the common grid. The cost estimate of those features cannot be represented so easily, but they are of crucial importance for the parameters of the grid and PSHPPs operation.
Methodology
The calculation of LCOE for PSHPP and SCGT includes the capital investment costs, operation and maintenance costs and different discount rates. Other LCOE elements such as pumping cost of PSHPP and fuel costs of SCGT were calculated under different scenarios.
PSHPP LCOE calculation
To calculate the PSHPP’s LCOE, the following equation is used:
where LCOE is levelized cost of electricity generated from PSHPP in $/kWh; I t , project investment costs needed to construct the PSHPP in the year t in $; M t , operation and maintenance cost in a certain year t excluding pumping cost in $; P t , pumping costs in the year t in $; E t , the electricity generation in the year t in kWh; r , discount rate; and n , expected PSHPP lifetime in years.
In general, the term \(\mathop \sum \nolimits_{t = 1}^{n} \frac{{I_{{t + M_{t} + P_{t} }} }}{{(1 + r)^{t} }}\) refers to the discounted project lifetime costs, while the term \(\mathop \sum \nolimits_{t = 1}^{n} \frac{{E_{t} }}{{(1 + r)^{t} }}\) represents the discounted project lifetime generated energy.
According to Eq. ( 1 ), the main elements required to calculate PSHPP’s LCOE during its lifetime are:
Capital investment costs.
Operation and maintenance costs (O&M).
Pumping cost.
Discount rate ( r ).
Capital investment costs
The investment costs are those costs needed to construct PSHPP including all capital costs (overnight costs) and the required investments to cover them. These costs are required mainly for: engineering, procurement and construction works of PSHPP including civil, mechanical and electrical works.
The breakdown of PSHPP capital costs follows the plant component decomposition:
Electromechanical system comprising turbines, actuators, regulators, generators, circuit breakers, a grounding system and a protection system;
Control and monitoring system including supervisory control and data acquisition (SCADA); and
Water supply system containing water depressing and drainage systems.
Other costs included in the capital investment cost are civil works, the cost of interconnecting the PSHPP to the grid and the water and piping system.
Civil works
Due to the topography of the site, SWECO International’s study (SWECO 1997 ) assumed this civil cost to count an amount of 25% of the total project capital cost. The tunnel cost is a very large part of the civil works; therefore, it is important to find the tunnel cross section that gives the lowest cost (Rognlien 2012 ). Optimization of the tunnel cross section was elaborated by SWECO (SWECO 1997 ); for further details, refer to (SWECO 1997 ). The cost of interconnecting the PSHPP to the grid:
Based on data from Egyptian Electricity Transmission Company, the average cost of a 500 V three phase transmission line is 0.4 M$/km with a capacity of 1750 MVA. The 2100 MW PSHPP will need three transmission lines to be able to absorb/evacuate electricity from/to the Egyptian Unified Grid. Another extra bundle of three phase transmission line type is assumed to be interconnected with the PSHPP for emergency. The nearest point to the Egyptian Unified Grid that the PSHPP can be interconnected is at a distance of 8 km. According to these assumptions, the total interconnection cost in the study will amount to 12.8 M$.
Water and piping system
PSHPP will need a large volume of water at the start-up, to run the PSHPP with its full power capacity. This water volume amounts to 9 mcm (SWECO 1997 ). This volume acts as an active volume of the upper reservoir, bearing in mind that there will be daily evaporation, which can be calculated according to the annual evaporation rate in Suez (3400 mm/year) (Edgell 2006 ). The leakage expected during operation was also taken into account, so the total daily backup water amount is assumed to be 10 4 m 3 /day.
The total cost of water needed for operating of the PSHPP is calculated according to Eq. ( 2 ):
where water cost is cost of water required to fill in the upper reservoir during a certain number of years in $; UR c , upper reservoir water capacity in m 3 ; W l , annual loss of water due to evaporation in m 3 ; N , the number of construction years discarding 1 year for filling the upper reservoir (4 years in this case); and P w , price of 1 m 3 of water in $.
PSHPP has a site specific capital investment cost, which is significantly variable from one site to another; the above costs are site specific to the area of Mount Attaqa in Egypt. This unpredictability in the capital investment costs is mainly due to the changing civil works costs, where the plant component cost variability is slight. In this study, the cost of plant components is assumed to be fixed at 1600 $/kW, while the civil works cost varies from 600 to 3600 $/kW at a fixed step of 200 $/kW to generalize the analysis. This assumed range is made to acquire the minimum and maximum possible values of LCOE of the PSHPP in Egypt based on the world’s lowest and highest figures of constructing such kind of power plants (2200–5200 $/kW including the costs of plant components). The cost of interconnecting the PSHPP to the grid and the cost of water and piping system per kW is included in the variable number of civil works to sum up the value of the capital investment cost.
All the above-mentioned costs were assumed as the total fixed capital costs required to completely construct the PSHPP; however, there are extra costs that will be incurred to secure financing to all these capital costs. The finance structure of the PSHPP was assumed to be divided into two debt loans as follows:
Loan from local banks
It amounts 40% of the total project capital costs to be repaid over 10 years with an annual interest rate of 11% and grace period 2 years with a commitment fee 0.5% on the remaining balance at each year during the construction period of the project.
Loan from foreign banks
It amounts 60% of the total project capital costs to be repaid over 20 years with an annual interest rate of 6% and grace period 3 years with a commitment fee 0.5% on the remaining balance at each year during the construction period of the project.
Operation and maintenance (O&M) costs
The O&M costs are those costs needed to ensure the proper operation of the PSHPP and are divided into fixed O&M costs and backup water costs.
Fixed O&M costs
These costs were assumed to be 30.8 $/kW annually according to Black and Vetch Consultancy Company projections report of performance and cost data for power plants (Black and Vetch 2012 ). These costs include the wages and salaries of the PSHPP operators and engineers as well as the spare parts and the refurbishment of mechanical and electrical equipment.
Backup water cost
As stated above, PSHPP will require backup water during PSHPP operation to recover the evaporated amounts and its cost is included in the operational costs. The cost of backup water is calculated using Eq. ( 2 ) taking into account that the number of years ( N ) will be 1 because this cost will be encountered annually. The upper reservoir water capacity UR c term in the equation will be equal to zero because the loss in water is only calculated. P w is assumed to be escalated annually during the lifetime of the PSHPP by 3% following the consumer price index (CPI) in USA which amounted 2.34–2.39% in 2015 (Trading Economics 2016 ).
Pumping cost
Pumping cost is the cost of electricity that will run the PSHPP in pumping mode to lift up water from the lower reservoir to the upper reservoir during off-peak times. This cost will differ based on the following scenarios (Fig. 5 ):
Pumping cost scenarios in combination with different investment/capital costs
First scenario
The pumping cost is assumed to be equal to the price of buying electricity from the Egyptian Unified Grid at off-peak time on the extra high voltage level (220 kV) and this cost will include capacity payments (1.54 $/kW Month) and energy payments (0.0248 $/kWh) (EgyptERA 2015 ).
Second scenario
The pumping cost is assumed to be 70% of the total energy payment cost in the first scenario (0.01736 $/kWh), while this percentage represents the average cost of fuel usage in electricity generation according to the Egyptian Electricity Holding Company (EEHC).
Third scenario
The pumping cost is assumed to be zero. This assumption is based on supplying electricity for pumping, from renewable power plants that are connected to grid and do not generate electricity during peak times, or from the available spinning reserve from the traditional thermal power plants during off-peak time.
The pumping cost is assumed to be escalated by 3% annually over the project lifetime in the first and second scenarios.
Discount rate
The discount rate is the opportunity cost of capital or in other words is the return on investments foregone elsewhere by committing capital to the project under consideration. Mathematically, it refers to the interest rate used to calculate the present value of future cash flow in a discounted cash flow (DCF) analysis. It is used to take into account not only the time value of money, but also the uncertainties and risks the cash flow may suffer from in the future; the higher the expected risks and uncertainties in the future, the higher the discount rate is expected. The future value of a cash flow at certain specific period (year, month or day) can be discounted using a discount factor (DF) which is the amount by which any future value must be multiplied to convert it into present value and it can be calculated using Eq. ( 3 ):
where DF is discount factor; t , number of periods (years) separating the future and present value; and r , the discount rate.
In some studies, the discount rate used for a project is considered equal to the project weighted average cost of capital (WACC) and this is when discounting the cash flow from a utility or firm point of view. Typically, a utility finances its assets and projects either through debt, bank loans, bonds. WACC is the weighted average of costs related to financing a certain project from loans (Deloitte 2014 ).
The general formula used to determine the average cost of raising capital through debt (WACC) is:
where WACC is weighted average cost of capital; L LB , percentage of the loan from local banks; IR LB , annual interest rate on the loan from local banks; L FB , loan from foreign banks; IR FB , annual interest on the loan from foreign banks; and T c , corporate tax rate.
According to the WACC in Eq. ( 4 ) and based on the study assumptions, the PSHPP project is totally financed by two debt loans. The corporate tax is assumed zero because such kind of power plants are normally executed by the governmental sector. According to this, the WACC value 8% is assumed to be the discount rate of the study for both PSHPP and SCGT LCOE.
From a national point of view, the discount rate value can be equal to the country’s discount rate which includes all financial risks related to the country, such as credit rating and risk premium. The Central Bank of Egypt (CBE) announced in 2015 a discount rate of 9.75% (almost 10%). Consequently, r values of 10%, besides the 8%, as well as two other values of 12 and 14%, representing pessimistic cases, were taken into consideration. It should be noted that a higher risk may be encountered because PSHPP has a long lifetime (over 60 years).
General assumptions
There are other assumptions that have been taken into account in calculating LCOE for PSHPP including its lifetime which is assumed to be 60 years (IRENA 2012 ), supposing the operation of the PSHPP to start at the end of the year 2020 taking 5 years as a construction period for PSHPP. The PSHPP is expected to deliver energy for 10 h a day (EBASCO 1993 ). This assumption is for design purposes.
The forced outage rate, which is the shutdown condition of a power station due to unexpected breakdown is assumed to be 5% (Thapar 2002 ) and the scheduled maintenance period is 20 days a year; therefore, the availability factor of the PSHPP is calculated using Eq. ( 5 ) (34.1%).
where AF is availability factor of PSHPP; H d , expected operating hours of PSHPP per day; FOR, expected forced outage rate; and N d , number of days of scheduled maintenance.
Therefore, the total annual electrical energy ( E t ) generated is calculated (6274 GWh) using Eq. ( 6 ).
where E t is annual energy generated; P , output power capacity; and AF, availability factor of PSHPP.
On the other side, the electrical energy needed to pump up water to the upper reservoir is calculated as 8375 GWh (Eq. 7 ), assuming PSHPP typical cycle efficiency 0.75.
where η is cycle efficiency; E t , annual generated energy from PSHPP; and E p , annual energy needed to pump up water.
This is a simplified representation. It should be noted that in this connection, several lines of financial project development are running parallel: design, administration and permits, construction, supply and installation of equipment, and more complicated financing processes are included.
LCOE calculations for SCGT
The formula used for calculating the LCOE of SCGT power plant is:
where all the terms of the equation are similar to Eq. ( 1 ) except F t which represents the fuel costs in the year t in $.
According to Eq. ( 8 ), the main components required to calculate LCOE for SCGT power plant during its whole lifetime are:
Fuel costs.
Discount rate.
These components are clarified below in detail with explanation to the scenarios applied for fuel cost as one of the main affecting components on the LCOE of SCGT power plant. Within each scenario for fuel cost, there will be only one value for LCOE (Fig. 6 ).
Fuel cost scenarios applied to conduct LCOE value of each traditional generation technology
Capital investment cost
Capital investment cost of SCGT power plant does not vary much from one site to another within the same country, in contrast to capital investment cost of PSHPP which is site specific. Therefore, one average value for capital investment cost of SCGT will be used in the study which is 968 $/kW. This value, according to the Annual Energy Outlook 2015 (EIA 2013 ), represents an average for utility scale SCGT power plants electricity projects.
The above-mentioned capital investment cost is the overnight costs required to completely construct an SCGT power plant and this cost is assumed to be financed by two debt loans as follows:
It amounts 20% of the total project capital costs to be repaid over 10 years with an annual interest rate of 11% and grace period 2 years with a commitment fee 0.5% on the remaining balance at each year during the construction period of the project.
It amounts 80% of the total project capital costs to be repaid over 20 years with an annual interest rate of 6% and grace period 3 years with a commitment fee 0.5% on the remaining balance at each year during the construction period of the project. A different financing structure for SGT from that of PSHPP (60% foreign loan + 40% local loan) is assumed, due to the fact that PSHPP construction has many local works such as civil infrastructure, drillings and piping systems.
The O&M costs can be classified into fixed O&M and non-fuel variable O&M. The fixed O&M cost includes all costs that are independent of the electricity generation production of the SCGT power plant such as wages. The annual fixed cost for SCGT used in this study is 7.34 $/KW (EIA 2013 ). The non-fuel variable O&M cost includes all costs that are dependent on the electricity production level such as equipment outage maintenance, utilities and consumables such as chemicals. According to (EIA 2013 ), the variable cost used in calculating LCOE of SCGT is 15.44 $/MWh.
Fuel prices are one of the key factors that affect the LCOE of the SCGT power plant when compared to LCOE of PSHPP. Natural gas (NG) is the main fuel used for electricity generation in SCGT power plant, while light fuel oil (LFO) is the emergency fuel used in the case of unavailability of NG. It is assumed that the fuel used in SCGT is 90% NG, while the residual 10% is LFO in case of emergencies. NG and LFO can be provided through local resources in Egypt or through import. If the government continues to subsidize local fuel prices supplied to the electricity sector in Egypt, it is expected that there will not be an economic competitiveness of PSHPP over SCGT. According to the Egyptian Government, by 2020 subsidies on fuel prices will be completely eliminated. Since 2016, fuel prices have been doubled and more increase is expected by the next few years. Thus, the realistic economic situation is represented using international fuel prices. Two scenarios of international fuel prices in calculating the LCOE of SCGT are studied:
International fuel prices are assumed fixed over the different technologies lifetime based on year 2014 prices for NG and year 2015 prices for LFO.
International fuel prices are assumed to be annually escalated by 3% over the different technologies lifetime based on year 2015 prices.
The international fuel price of NG was 8.22 $/MBTU in 2014 according to ICIS Heren Energy Ltd. (ICIS 2016 ). NG drastically dropped to its lowest levels in 2015 since 1999 and such a drop in price was assumed as an odd situation due to political issue. So calculations of LCOE of SCGT were based on the 2014 price of NG, while the average international price of LFO was 12 $/MBTU in 2015. The subsidized local prices of NG and LFO were only 37 and 43% of their international prices, in 2015 (Insee 2016 ). There are many factors that can affect the amount of fuel consumed by technology to generate electricity; some of these factors are the fuel type represented in its heating value and the efficiency of the technology or power plant represented in its heat rate (EIA 2016 ). The amount of fuel needed to generate a specific amount of electricity (kWh) from fossil fuel fired power plant is calculated using Eq. ( 9 ) as follows:
where FA is amount of fuel needed to generate a specific amount of electricity in m 3 ; HR, heat rate of fossil fuel fired power plant in BTU/kWh; HC, fuel heat content in BTU/m 3 for NG and in BTU/kg for LFO; and E t , the generated electricity in kWh. The heating values used in the study are 33,723 BTU/m 3 for NG and 41,960 BTU/kg for LFO, while the average heat rate of SCGT is 10,783 BTU/kWh according to data available from EEHC (EEHC 2015 ).
Similar to that of the PSHPP, the values of r were assumed to be 8, 10, 12 and 14%. All the study calculations will be in foreign currency US dollars ($) using the exchange rate to convert between the local currency in Egyptian pounds (L.E.) and US dollars as 10 L.E./$. This exchange rate is assumed to be fixed over the project lifetime and is applied at the start of year 2015, which is the base year for the study.
The currency exchange rate may have a significant effect on the project capital cost during the construction period and a slight effect during the PSHPP operation. This is because the components that will be imported from outside using foreign currencies for construction and maintenance purposes during process of installation. Accordingly, contingencies and escalation rates were taken into consideration to overcome such exchange rate issues. The escalation rates in O&M during the project lifetime were assumed to be 3% annually. Thus, the assumption of 3% as escalation rate in O&M is a reasonable assumption for all O&M costs of PSHPP and SCGT and for pumping cost too.
For each scenario and specific discount rate (8, 10, 12 and 14%), the relation between the LCOE of PSHPP and the capital investment cost ranging between 2200 and 4200 $/kW is fitted through regression (using least square method). The LCOE of SCGT is to be used to determine the competitiveness range allowed for PSHPP’s capital investment costs. The relationship is fitted such that the investment cost is the dependent variable, while the LCOE is the independent variable.
Table 1 shows a summary of all the assumptions required for the calculations.
Results of calculating the LCOE for PSHPP under three scenarios after applying different discount rates, as a function of investment capital cost, are plotted in Figs. 6 , 7 and 8 . It is clear from the figures that the relation is linear. To obtain the values of investment cost at which PSHPP is competitive to SCGT, the relationships have been expressed as a linear function through application of regression technique (Table 2 ).
LCOE of PSHPP versus variable capital cost at different discount rates (Scenario 1)
LCOE of PSHPP versus variable capital cost at different discount rates (Scenario 2)
Table 3 shows LCOE of SCGT at different discount rates in case of there is no increase in international fuel costs and in case of escalated international fuel costs.
Pumping cost scenario 1
Adopting the case that pumping cost is equal to the price of buying electricity from the Egyptian Unified Grid at off-peak time, when substituting by values in Table 3 with the corresponding regression equation in Table 2 it is found that PSHPP has competitiveness over SCGT power plants only in the escalated international fuel costs fuel scenario at r 8, 10 and 12%, provided that the capital cost for PSHPP should not exceed 2680.95, 2415.298 and 2211.146 $/kW, respectively. These milestones are shown clearly in Fig. 7 on the curves of different discount rates and it is noticed that on the curve ( r 8%) there is a tolerance of the capital cost of the PSHPP to range from 2200 $/kW (minimum capital cost of any PSHPP assumed in this study) to 2679 $/kW, while on the curve where r is assumed to be 12% the tolerance is very tight (2200–2222 $/kW).
PSHPP’s LCOE does not have any competitiveness over that of SCGT in the escalated international fuel cost scenario as a whole, and at r 14% in the fixed fuel cost scenario (the points of intersection are out of PSHPP’s capital cost range).
Pumping cost scenario 2
Take the assumption that the pumping cost is 70% (fuel portion cost) of the total energy payment cost in first scenario. When checking the possibility of intersection between LCOE values of PSHPP and those of SCGT (Fig. 8 ), it is found that PSHPP has competitiveness over SCGT power plants still only in the case of escalated international fuel costs, but in this scenario the range of the possibility of the capital cost is more flexible; also, its competiveness at r 14% exists. PSHPP has competitiveness over SCGT power plants at r 8, 10, 12 and 14% (Table 3 ), on condition that the capital cost for PSHPP should not exceed 3136.82, 2780.692, 2522.222 and 2325.425 $/kW, respectively.
Pumping cost scenario 3
From the intersection between LCOE values of PSHPP with those of SCGT (Fig. 9 ), it is found that at both fuel cost scenarios and all discount rates, PSHPP will have competitiveness over SCGT. For the fixed fuel cost scenario, the LCOE of PSHPP is more economical than that of SCGT provided that its capital cost does not exceed 2938.555, 2616.615, 2392.65 and 2226.872 $/kW at r 8, 10, 12 and 14%, respectively. For the pessimistic fuel cost, its capital cost should not exceed 4179.67, 3629.11, 3246.991 and 2968.07 $/kW for the same discount rates. This is an upgrade in competiveness from the above both scenarios, and the range of tolerance of PSHPP capital cost in the escalated fuel cost scenario reached about 4180 $/kW.
LCOE of PSHPP versus variable capital cost at different discount rates (Scenario 3)
In this manuscript, a simplified approach to define the conditions at which PSHPP has competitiveness over SCGT power plants. PSHPPs have many additional features of their operation in the common grid whose cost estimate cannot be represented so easily.
It can be concluded from the results that PSHPP does not have an absolute economic competitiveness compared with SCGT power plant, but the economic viability of constructing PSHPP is variable according to different factors and conditions. From the perspective of electricity sector in Egypt, as long as the government subsidizes the fuel prices supplied to SCGT power plants, there will be no need to construct PSHPP in Attaqa Mountain.
The pure economic competitiveness of PSHPP over SCGT power plant will appear in case of using the international fossil fuel prices and taking into account the huge amounts of foreign currency to import such fossil fuels to cover the domestic demand from fuel in other sectors than electricity sector. This competitiveness of PSHPP can be increased also by decreasing the pumping cost to lift water up in PSHPP, which is a strategic decision by the government. PSHPP competitiveness over SCGT technology increases more when the limitations and conditions at which installing PSHPP in Egypt are more relaxed. Therefore, ideally, there should be a double tariff structure mechanism designed to get the advantages of PSHPPs as a clean energy source to maximize its benefits.
Barbour, E., Wilson, I. G., Radcliffe, J., Ding, Y., & Li, Y. (2016). A review of pumped hydro energy storage development in significant international electricity markets. Renewable and Sustainable Energy Reviews, 61, 421–432.
Article Google Scholar
Baxter, R. (2006). Energy storage: A nontechnical guide . Houston: PennWell Books.
Google Scholar
Black & Vetch Consultancy Company. (2012). Cost and performance data for power generation technologies prepared for the national renewable energy laboratory, Cost Report, February 2012. https://www.bv.com/docs/reports-studies/nrel-cost-report.pdf . Accessed 2016.
Breeze, P. (2014). Chapter 10: Power system energy storage technologies. In Power generation technologies (pp. 195–221). Oxford: Newnes.
Deloitte. (2014). Some common mistakes to avoid in estimating and applying discount rates, A middle east point of view. Discount rates report. https://www2.deloitte.com/content/dam/Deloitte/xe/Documents/About-Deloitte/mepovdocuments/mepov13/dtme_mepov13_Discount%20rates.pdf . Accessed 2016.
Ebasco Overseas Corporation. (1993). Feasibility study of a pump storage hydroelectric power station, Egypt, Final Phase 1 Report, September 1993.
Edgell, H. S. (2006). Arabian deserts: Nature, origin and evolution . Netherlands: Springer.
Book Google Scholar
Egyptian Electric Utility and Consumer Protection Regulatory Agency (EgyptERA). (2015). Electricity tariff [Internet]. www.Egyptera.org . Accessed 2016.
Egyptian Electricity Holding Company (EEHC). (2015). Annual report 2014/2015 [Internet]. Arab Republic of Egypt, Ministry of Electricity and Renewable Energy. http://www.moee.gov.eg/english_new/EEHC_Rep/2014-2015en.pdf . Accessed 2016.
ICIS. (2016). Natural gas prices, markets & analysis [Internet], ICIS. https://www.icis.com/ . Accessed 2016.
Insee. (2016). International prices of imported new materials [Internet]. National Institute of Statistics and Economic Studies. https://www.bdm.insee.fr/bdm2/affichageSeries?idbank=001642883&page=tableau&request_locale=en . Accessed 2016.
International Electro-Technical Commission (IEC). (2011). Electrical energy storage—White paper, Geneva, Switzerland. http://www.iec.ch/whitepaper/energystorage/ . Accessed 2016.
International Energy Agency (IEA). (2014). Technology roadmap energy storage [Internet]. France: OECD/IEA. http://www.iea.org/publications/freepublications/publication/technologyroadmapenergystorage.pdf . Accessed 2016.
International Renewable Energy Agency (IRENA). (2012). Renewable energy technologies: cost analysis series. Hydropower [Internet]. Power Sector, June 2012. Report No. 1(3/5). https://www.irena.org/documentdownloads/publications/re_technologies_cost_analysis-hydropower.pdf . Accessed 2016.
Kaplan, S. (2008). Power plants: Characteristics and costs [Internet]. Congressional Research Services. Report No. RL34746. https://fas.org/sgp/crs/misc/RL34746.pdf . Accessed 2016.
Our World of Energy (OWOE). (2014). Other renewables energy—What is pumped hydroelectric storage? [Internet]. Our World of Energy. http://www.ourworldofenergy.com/vignettes.php?type=other-renewables-energy&id=10 . Accessed July 2014.
Rognlien, L. (2012). Pumped storage development in Ovre Otra, Norway, Norwegian University of Science and Technology. https://daim.idi.ntnu.no/masteroppgaver/007/7996/masteroppgave.pdf .
Sandia National Laboratories. (2016). DOE global energy storage database [Internet]. United States of America: Office of Electricity Delivery & Energy Reliability. www.energystorageexchange.org . Accessed 2016.
Swedish Consultants (SWECO). (1997). Mount Attaqa pumped storage project feasibility study. Final report, Stockholm, Sweden.
Thapar, O. D. (2002). Chapter 2: Techno-economic studies for capacity and unit size of hydro electric schemes. In Modern hydroelectric engineering practice in India: Electro-mechanical works (pp. 13–52). http://www.iitr.ac.in/departments/AH/uploads/File/Modern_Hydroelectric_Engineering_Practice_Prof_OD_Thapar/Volume_I/Chapter-2_Techno-Economic_Studies_for_Capacity_and_Unit_Size_of_Hydro_Electric_Schemes.pdf . Accessed 15 July 2016.
Trading Economics. (2016). United States Consumer Price Index (CPI) [Internet]. Trading Economics. http://www.tradingeconomics.com/united-states/consumer-price-index-cpi . Accessed 2016.
U.S. Energy Information Administration (EIA). (2013). Updated capital cost estimates for utility scale electricity generating plants [Internet]. United States of America: U.S. Department of Energy. Report No. 20585. http://www.eia.gov/outlooks/capitalcost/pdf/updated_capcost.pdf . Accessed 2016.
U.S. Energy Information Administration (EIA). (2016). How much coal, natural gas, or petroleum is used to generate a kilowatthour of electricity? [Internet]. United States of America: U.S. Department of Energy. https://www.eia.gov/tools/faqs/faq.cfm?id=667&t=6 . Accessed 2016.
Download references
Authors’ contributions
DA took part in compiling the required data, conducting the literature review, programming the mathematical model, verifying its accuracy, running the program, plotting the results, writing some parts of the paper and placing it in the required format for publication. RA determined the methodology and selected the research tools, and was involved in organizing the scenarios required to simulate, analyzing the results, code error debugging, results verification, reviewing the paper in terms of form and content, writing some parts and following up the publication of the paper. AMI proposed the research topic and general approach, reviewed the methodology and approved the scenarios to be simulated and verified the results and reviewed language and terminology used in the text. EAE performed approving the research topic and general approach, reviewing and verification of the results, and final review of language and terminology used in the text. All authors read and approved the final manuscript.
Competing interests
The authors declare that they have no competing interests.
Availability of data and materials
All data and materials are presented in the main paper.
Not applicable.
Publisher’s Note
Springer Nature remains neutral with regard to jurisdictional claims in published maps and institutional affiliations.
Author information
Authors and affiliations.
Egyptian Electricity Holding Company (EEHC), Ministry of Electricity and Renewable Energy, Cairo, Egypt
Diaa Abdellatif
National Water Research Center (NWRC), Ministry of Water Resources and Irrigation, El Qanatir, Egypt
Rameen AbdelHady
Electrical Power and Machines Department, Faculty of Engineering, Cairo University, Giza, Egypt
Ahmed M. Ibrahim & Essam Abu El-Zahab
You can also search for this author in PubMed Google Scholar
Corresponding author
Correspondence to Rameen AbdelHady .
Rights and permissions
Open Access This article is distributed under the terms of the Creative Commons Attribution 4.0 International License ( http://creativecommons.org/licenses/by/4.0/ ), which permits unrestricted use, distribution, and reproduction in any medium, provided you give appropriate credit to the original author(s) and the source, provide a link to the Creative Commons license, and indicate if changes were made.
Reprints and permissions
About this article
Cite this article.
Abdellatif, D., AbdelHady, R., Ibrahim, A.M. et al. Conditions for economic competitiveness of pumped storage hydroelectric power plants in Egypt. Renewables 5 , 2 (2018). https://doi.org/10.1186/s40807-018-0048-1
Download citation
Received : 26 November 2017
Accepted : 21 February 2018
Published : 01 March 2018
DOI : https://doi.org/10.1186/s40807-018-0048-1
Share this article
Anyone you share the following link with will be able to read this content:
Sorry, a shareable link is not currently available for this article.
Provided by the Springer Nature SharedIt content-sharing initiative
- Pumped storage hydroelectric power plant
- Levelized cost of electricity
- Simple cycle gas turbine
- On-peak demand
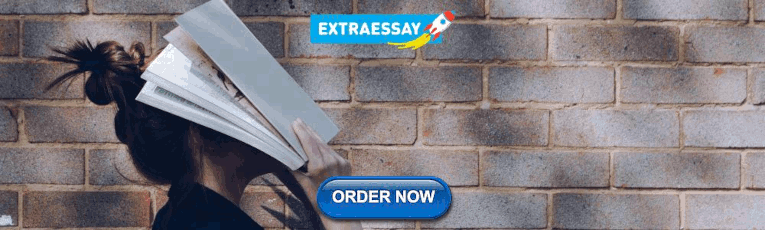
IMAGES
VIDEO
COMMENTS
Abstract. Hydroelectric power generation is one of many ways in which electricity can be generated. In 2009, the three most heavily used sources for generating electricity were coal, natural gas ...
This paper presented research small- and mini-hydroelectric power plants as an effective source of compensation for instability of non-guaranteed renewable energy sources (RES) power plants. HPPs play the main role for balancing the power system operation mode. To somehow compensate for the growth of RES generation in the power system, we can forced to start hydropower plants as a consumer in ...
This paper discusses how the hydropower sector needs to not only focus on energy production but also, include the negative social and environmental externalities caused by dams and recognize the unsustainability of current common practices. ... Alternating current then made possible the first hydropower plant at Redlands Power Plant, California ...
A hydro-power plant harnesses the energy of moving water to drive a turbine, which in turn will run a generator for electricity production. This technology is well understood and has many advantages (Breeze, 2005 ), among which a relatively low-marginal-cost and low greenhouse gas emission (Stoll et al., 2017 ), with nearly constant prices over ...
At facilities called hydroelectric power plants, hydropower is generated. Some power plants are l ocated. on rivers, streams, and canals, but for a reliable water supply, dams are needed. Dams ...
Adhau et al. [1] highlighted that micro-hydropower plants are emerging as a major renewable energy resource today as they do not encounter the problems of population displacement and environmental problems associated with the large hydro-power plants. In this paper the work is carried out for evaluating micro-hydro-power plants (MHPP) generation availability that can be applied to generation ...
technology, hydropower provides 16,4% of global electricity production. [7] As to the energy. storage the storage hydropower (including pumpe d storage, PS) represents between 96 - 99%. of the ...
0.4 m height had 13.92% efficiency at a water flowrate of 0.2 m3/s. Increasing the turbine's height and diameter between 0.5 and 0.7 m creates more blade surface area. With more blade surface area, the turbine can handle a greater force and flow speed of the vortex [21]. This increases the efficiency of the system.
The proposed methodology was built on three systems engineering and project complexity frameworks, and lessons learned from four case studies. Its contribution to hydropower development is related to (i) the incorporation of systems evolution over time on the development process, (ii) the identification and management of relationships among the ...
The paper discusses recent hydropower optimization research and development activities using metaheuristic approach. ... wind and hydro power plant (Acakpovi et al., 2015, ... from previous research very few of these researches are accessible for optimal short-run activity of hydroelectric power stations. Hence more research is needed in this ...
Hydroelectricity, which is defined as green energy, is a type of renewable energy. Despite the advantages of hydroelectric power plants, there are also negative effects caused by them. While power plants do provide advantages such as flood reduction, disaster management, mitigation of the effects of global warming and climate change; they can also lead to some unwanted environmental effects at ...
Their results suggest that the major share of the total theoretical potential for hydropower (58 PWh/year) is either already installed, not feasible or not profitable, leaving only 5.3 PWh/year as ...
Hydropower is an important renewable energy resource worldwide. However, its development is accompanied with environmental and social drawbacks. Issues of degradation of the environment and climate change can negatively impact hydropower generation. A sustainable hydropower project is possible, but needs proper planning and careful system design to manage the challenges. Well-planned ...
Hydropower, which is the most extensively used renewable energy, is sensitive to the change of streamflow under the great impact of precipitation. According to the relationship between the hydropower station generation and local precipitation, the impact of precipitation on hydropower can be analyzed. In this paper, the global climate model and regional climate model simulations in ...
Feature papers represent the most advanced research with significant potential for high impact in the field. A Feature Paper should be a substantial original Article that involves several techniques or approaches, provides an outlook for future research directions and describes possible research applications. ... CVA owns 32 hydroelectric power ...
1Mohit Jambu, 2Kulvir Singh, 3Bharat Bhushan. Abstract: Hydropower plays a crucial role within the development of the country because it provides power at cheaper rate being perpetual and renewable sources of energy. In hydroelectric power station, the energy of water is employed for generating electricity.
Hydropower is a reliable, clean, and efficient alternative to conventional fossil fuels and other renewable energy sources. The hydro turbine is the core of a hydropower plant, and the proper maintenance and operation of all other components are essential for maximizing energy production. Besides electricity generation, hydropower plants play a critical role in storing irrigation and drinking ...
The problem of hydroelectric generation has been modeled through multistage stochastic programming (MSP). In an MSP, there is a stochastic process that represents uncertain factors (e.g., hydro inflows and/or other factors), and decisions are made throughout time in such a way that expected costs (or some other metric) are minimized [].Every time a decision is made, it need to take into ...
Abstract. Renewable energy is one of the best option for challenging situation to supply and demand of electricity. Out of all renewable energy sources, hydro gives effective results at different phase. The hydro power plant helps us to generate the electricity from the potential energy of water. Site selection and huge investment are major ...
Feature papers represent the most advanced research with significant potential for high impact in the field. ... Turbine wear caused by hydro-abrasion due to suspended sediment in the turbine water has a negative impact on the power production and revenue of hydropower schemes. ... Currently, many such power plants are being upgraded with ...
As of 2020, when the hydroelectric power plants (HEPPs) in our country are classified according to their installed power, it is seen that there is 28571 MW for 683 hydroelec-tric power plants in operation, 3636 MW for 47 power plants under construction, and 15,955 MW for 526 plants under development (DSİ 2020).
Fig. 1 shows how the water output of a hydropower plant is the input of the second power plant and how the volume of water behind the dam and the overflow of dams, local flows, water output of dams, average and final water height are related to each other. This causal structure reflects the internal relationships between the various components and can help to understand the behavior of the system.
One of the EES technologies is pumped hydro storage. In 2011, the International Hydro Power Association (IHA) estimated that pumped hydro storage capacity to be between 120 and 150 GW (IRENA 2012) with a central estimate of 136 GW.In 2014, the total installed capacity of pumped storage hydroelectric power plants (PSHPPs) around the world reached 140 GW, which is very large compared to other ...