Springer Professional
Journal of nanoparticle research.
An Interdisciplinary Forum for Nanoscale Science and Technology
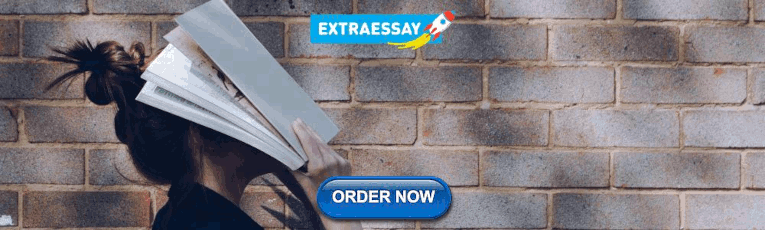
Current Publications
Journal of nanoparticle research 4/2024, journal of nanoparticle research 3/2024, journal of nanoparticle research 2/2024, journal of nanoparticle research 1/2024, journal of nanoparticle research 12/2023, journal of nanoparticle research 11/2023, journal of nanoparticle research 10/2023, journal of nanoparticle research 9/2023, journal of nanoparticle research 8/2023, journal of nanoparticle research 7/2023.
scroll for more
use your arrow keys for more
scroll or use arrow keys for more
About this journal
The Journal of Nanoparticle Research is a monthly peer-reviewed journal that explores the specific concepts, properties, phenomena and processes of structures at the nanoscale.
Coverage includes synthesis, assembly, transport, reactivity, and stability, and emphasizes realization and application of systems, structures and devices with novel functions obtained via precursor nanoparticles. The Journal fosters the interdisciplinary dissemination of knowledge by encouraging synergetic approaches originating from a wide range of disciplines, such as Physics, Chemistry, Biology and Health Care.
Perspectives now available for free online Perspective articles have a wide breadth of appeal because they evaluate research, industrial and societal trends centered around nanotechnology. See the bigger picture!
Premium Partners
- Business IT + Informatics
- Construction + Real Estate
- Electrical Engineering + Electronics
- Energy + Sustainability
- Insurance + Risk
- Finance + Banking
- Management + Leadership
- Marketing + Sales
- Mechanical Engineering + Materials
- Start single access now
- Access for companies
Journal of Nanoparticle Research Latest Publications
Total documents, published by springer-verlag.
- Latest Documents
- Most Cited Documents
- Contributed Authors
- Related Sources
- Related Keywords
Polymeric nanoparticles as therapeutic agents against coronavirus disease
The technology for improving stability of nanosuspensions in drug delivery, bioactive nano-selenium antagonizes cobalt nanoparticle-mediated oxidative stress via the keap1-nrf2-are signaling pathway.
AbstractAt present, no effective treatment exists for the clinical toxicity of cobalt nanoparticles (CoNPs, 30 nm) after metal-on-metal (MOM) artificial joint replacement. As such, a better understanding of the CoNPs-toxicity mechanism is necessary and urgent for the development of effective and safe detoxification drugs. Our purpose was to explore the role of bioactive nano-selenium (BNS, > 97%) in antagonizing the toxicity of CoNPs and its mechanism through the Keap1-Nrf2-ARE signaling pathway. To examine BNS detoxification, we exposed HUVEC cells to CoNPs and BNS for 24 h, before measuring cell activity, reactive oxygen species (ROS), the GSH level, inflammatory factors, and KNA signaling pathway-related transcript and protein expression. CoNPs stimulate intracellular inflammation and ROS production to bring about significant downregulation of cellular activity and the GSH level. Conversely, BNS reduces ROS generation and suppresses inflammatory factors within cells to reduce CoNPs-mediated cytotoxicity, possibly via the KNA signaling pathway. Based on our results, BNS antagonizes CoNPs toxic effects by suppressing ROS production through the KNA pathway. Our research provides new insight into the clinical treatment of CoNPs toxicity and explores the potential of BNS in detoxification therapy. Trial registration: no human participant.
Hydrated zirconia nanoparticles as media for electrical charge accumulation
A mn-doped calcium phosphate nanoparticle-based multifunctional nanocarrier for targeted drug delivery and cellular mr imaging, palladium nanoparticles supported on aluminum oxide (al2o3) for the catalytic hexavalent chromium reduction, a novel ag nanoparticles purification method and the conductive ink based on the purified ag nanoparticles for printed electronics, use of magnesium nanomaterials in plants and crop pathogens, cu2o/cuo heterojunction formed by thermal oxidation and decorated with pt co-catalyst as an efficient photocathode for photoelectrochemical water splitting, vapor–liquid-solid silicon wires’ synthesis catalyzed by a low-surface tension post-transition metal: effect of process parameters, export citation format, share document.
- English Editing
- Translation
- Figure Services
- Poster Preparation
- Video Abstracts
- My Searches
- My Journals
Journal of Nanoparticle Research
Aims and scope.
The objective of the Journal of Nanoparticle Research is to disseminate knowledge of the physical, chemical and biological phenomena and processes in structures that have at least one lengthscale ranging from molecular to approximately 100 nm (or submicron in some situations), and exhibit improved and novel properties that are a direct result of their small size. Nanoparticle research is a key component of nanoscience, nanoengineering and nanotechnology. For the full aims and scope, please visit the journal website.
Responsiveness Not Provided
Publication speed not provided, submission fees $, publication or page charges $.
None listed
Color charges
Online publication of color illustrations is free of charge. For color in the print version, authors will be expected to make a contribution towards the extra costs.
Open Access Policy
Open access available yes.
Journal complies with funding agency agreements. In addition, authors have the option to make their article available to non-subscribers immediately upon publication via Springer Open Choice (Typical cost: US $3,000).
Verified journal
This journal is included in the JournalGuide whitelist of reputable titles. Learn more here.
General information
Journal profile.
This journal's profile page has not been yet been claimed by an official representative.
More actions
Journal common names.
Do you know this journal by another common name or abbreviation? Enter it here and use it for your future searches

An official website of the United States government
The .gov means it’s official. Federal government websites often end in .gov or .mil. Before sharing sensitive information, make sure you’re on a federal government site.
The site is secure. The https:// ensures that you are connecting to the official website and that any information you provide is encrypted and transmitted securely.
- Publications
- Account settings
Preview improvements coming to the PMC website in October 2024. Learn More or Try it out now .
- Advanced Search
- Journal List
- Front Microbiol
- PMC10168541
A review on nanoparticles: characteristics, synthesis, applications, and challenges
The significance of nanoparticles (NPs) in technological advancements is due to their adaptable characteristics and enhanced performance over their parent material. They are frequently synthesized by reducing metal ions into uncharged nanoparticles using hazardous reducing agents. However, there have been several initiatives in recent years to create green technology that uses natural resources instead of dangerous chemicals to produce nanoparticles. In green synthesis, biological methods are used for the synthesis of NPs because biological methods are eco-friendly, clean, safe, cost-effective, uncomplicated, and highly productive. Numerous biological organisms, such as bacteria, actinomycetes, fungi, algae, yeast, and plants, are used for the green synthesis of NPs. Additionally, this paper will discuss nanoparticles, including their types, traits, synthesis methods, applications, and prospects.
1. Introduction
Nanotechnology evolved as the achievement of science in the 21st century. The synthesis, management, and application of those materials with a size smaller than 100 nm fall under the interdisciplinary umbrella of this field. Nanoparticles have significant applications in different sectors such as the environment, agriculture, food, biotechnology, biomedical, medicines, etc. like; for treatment of waste water ( Zahra et al., 2020 ), environment monitoring ( Rassaei et al., 2011 ), as a functional food additives ( Chen et al., 2023 ), and as a antimicrobial agents ( Islam et al., 2022 ). Cutting-edge properties of NPs such as; nature, biocompatibility, anti-inflammatory and antibacterial activity, effective drug delivery, bioactivity, bioavailability, tumor targeting, and bio-absorption have led to a growth in the biotechnological, and applied microbiological applications of NPs.
A particle of matter with a diameter of one to one hundred nanometers (nm) is commonly referred to as a nanoparticle or ultrafine particle. Nanoparticles frequently exhibit distinctive size-dependent features, mostly due to their tiny size and colossal surface area. The periodic boundary conditions of the crystalline particle are destroyed when the size of a particle approaches the nano-scale with the characteristic length scale close to or smaller than the de Broglie wavelength or the wavelength of light ( Guo et al., 2013 ). Because of this, many of the physical characteristics of nanoparticles differ significantly from those of bulk materials, leading to a wide range of their novel uses ( Hasan, 2015 ).
2. Emergence of nanotechnology
Nanotechnology emerged in the 1980s due to the convergence of experimental advances such as the invention of the scanning tunneling microscope in 1981 and the discovery of fullerenes in 1985 ( Bayda et al., 2019 ), with the elucidation. The popularization of a conceptual framework for nanotechnology goals began with the publication of the book Engines of Creation in 1986 ( Bayda et al., 2019 ).
2.1. Early stage of NPs
Carbon nanotubes have been discovered in pottery from Keeladi, India, dating from around 600–300 BC ( Bayda et al., 2019 ; Kokarneswaran et al., 2020 ). Cementite nanowires have been discovered in Damascus steel, a material that dates back to around 900 AD; nevertheless, its origin and creation method are unclear ( Kokarneswaran et al., 2020 ). However, it is unknown how they developed or whether the material containing them was used on purpose.
2.2. Discovery of C, Ag, Zn, Cu, and Au nanoparticles
Carbon NPs were found in 1991, and Iijima and Ichihashi announced the single-wall carbon nanotube synthesis with a diameter of 1 nanometer in 1993 ( Chen et al., 2021 ). Carbon nanotubes (CNTs), also known as Bucky tubes, are a kind of nanomaterial made up of a two-dimensional hexagonal lattice of carbon atoms. They are bent one way and joined to produce a hollow cylindrical cylinder. Carbon nanotubes are carbon allotropes that fall between Fullerene (0 dimensional) and Grapheme (2 dimensional) ( Chen et al., 2021 ).
In addition, M. C. Lea reported that the synthesis of citrate-stabilized silver colloid almost 120 years ago ( Nowack et al., 2011 ). This process produces particles with an average diameter of 7 to 9 nm. Nanoscale size and citrate stabilization are analogous to recent findings on nanosilver production employing silver nitrate and citrate ( Majeed Khan et al., 2011 ). The use of proteins to stabilize nanosilver has also been documented as early as 1902 ( Nowack et al., 2011 ; Beyene et al., 2017 ). Since 1897, a nanosilver known as “Collargol” has been made commercially and used for medicinal purposes ( Nowack et al., 2011 ). Collargol, a type of silver nanoparticle, has a particle size of about 10 nanometers (nm). This was determined as early as 1907, and it was found that the diameter of Collargol falls within the nanoscale range. In 1953, Moudry developed a different type of silver nanoparticle called gelatin-stabilized silver nanoparticles, with a diameter ranging from 2–20 nm. These nanoparticles were produced using another method than Collargol. The necessity of nanoscale silver was recognized by the creators of nanosilver formulations decades ago, as seen by the following remark from a patent: “for optimal efficiency, the silver must be disseminated as particles of colloidal size less than 25 nm in crystallite size”( Nowack et al., 2011 ).
Gold NPs (AuNPs) have a long history in chemistry, going back to the Roman era when they were used to decorate glassware by staining them. With the work of Michael Faraday, who may have been the first to notice that colloidal gold solutions have characteristics different from bulk gold, the contemporary age of AuNP synthesis began more than 170 years ago. Michael Faraday investigated the making and factors of colloidal suspensions of “Ruby” gold in 1857. They are among the magnetic nanoparticles due to their distinctive optical and electrical characteristics. Under specific illumination circumstances, Faraday showed how gold nanoparticles might create solutions of various colors ( Bayda et al., 2019 ; Giljohann et al., 2020 ).
3. Classification of NPs
Nanoparticles (NPs) are categorized into the following classes based on their shape, size, and chemical characteristics;
3.1. Carbon-based NPs
Fullerenes and carbon nanotubes (CNTs) are the two essential sub-categories of carbon-based NPs. NPs of globular hollow cages, like allotropic forms of carbon, are found in fullerenes. Due to their electrical conductivity, high strength, structure, electron affinity, and adaptability, they have sparked significant economic interest. These materials have organized pentagonal and hexagonal carbon units, each of which is sp2 hybridized. While CNTs are elongated and form 1–2 nm diameter tubular structures. These fundamentally resemble graphite sheets rolling on top of one another. Accordingly, they are referred to as single-walled (SWNTs), double-walled (DWNTs), or multi-walled carbon nanotubes (MWNTs) depending on how many walls are present in the rolled sheets ( Elliott et al., 2013 ; Astefanei et al., 2015 ).
3.2. Metal NPs
Metal NPs are purely made of metals. These NPs have distinctive electrical properties due to well-known localized surface Plasmon resonance (LSPR) features. Cu, Ag, and Au nanoparticles exhibit a broad absorption band in the visible region of the solar electromagnetic spectrum. Metal NPs are used in several scientific fields because of their enhanced features like facet, size, and shape-controlled synthesis of metal NPs ( Khan et al., 2019 ).
3.3. Ceramics NPs
Ceramic NPs are tiny particles made up of inorganic, non-metallic materials that are heat-treated and cooled in a specific way to give particular properties. They can come in various shapes, including amorphous, polycrystalline, dense, porous, and hollow, and they are known for heat resistance and durable properties. Ceramic NPs are used in various applications, including coating, catalysts, and batteries ( Sigmund et al., 2006 ).
3.4. Lipid-based NPs
These NPs are helpful in several biological applications because they include lipid moieties. Lipid NPs typically have a diameter of 10–1,000 nm and are spherical. Lipid NPs, i.e., polymeric NPs, have a solid lipid core and a matrix consisting of soluble lipophilic molecules ( Khan et al., 2019 ).
3.5. Semiconductor NPs
Semiconductor NPs have qualities similar to metals and non-metals. That is why Semiconductor NPs have unique physical and chemical properties that make them useful for various applications. For example, semiconductor NPs can absorb and emit light and can be used to make more efficient solar cells or brighter light-emitting diodes (LEDs). They can make smaller and faster electronic devices, such as transistors, and can be used in bio imaging and cancer therapy ( Biju et al., 2008 ).
3.6. Polymeric NPs
Polymeric NPs with a size between 1 and 1,000 nm can have active substances surface-adsorbed onto the polymeric core or entrapped inside the polymeric body. These NPs are often organic, and the term polymer nanoparticle (PNP) is commonly used in the literature to refer to them. They resemble Nano spheres or Nano capsules for the most part ( Khan et al., 2019 ; Zielińska et al., 2020 ).
4. Types of different metal-based NPs
Metal NPs are purely made of metal precursors. Due to well-known localized surface plasmon resonance (LSPR) characteristics, these NPs possess unique optoelectrical properties. NPs of the alkali and noble metals, i.e., Cu, Ag, and Au, have a broad absorption band in the visible zone of the solar electromagnetic spectrum. The facet, size, and shape-controlled synthesis of metal NPs are essential in present-day cutting-edge materials ( Dreaden et al., 2012 ; Khan et al., 2019 ).
4.1. Silver nanoparticles (AgNPs)
AgNPs are particles with a size range of 1–100 nanometers made of silver. They have unique physical and chemical properties due to their small size, high surface area-to-volume ratio, and ability to absorb and scatter light in the visible and near-infrared range. Because of their relatively small size and high surface-to-volume ratios, which cause chemical and physical differences in their properties compared to their bulk counterparts, silver nanoparticles may exhibit additional antimicrobial capabilities not exerted by ionic silver ( Shenashen et al., 2014 ).
Besides, AgNPs can be created in various sizes and forms depending on the manufacturing process, the most common of which is chemical reduction. The AgNPs were created by chemically reducing a 12 mM AgNO3 aqueous solution. The reaction was carried out in an argon environment using 70 mL of this solution containing PVP (keeping the molar ratio of the repeating unit of PVP and Ag equal to 34) and 21 mL of Aloe Vera. The mixture was agitated in ultrasonic for 45 min at ambient temperature, then heated 2°C/min to 80°C and left for 2 h to generate a transparent solution with tiny suspended particles that must be removed by simple filtering ( Shenashen et al., 2014 ; Gloria et al., 2017 ).
4.2. Zinc nanoparticles (ZnONPs)
Zinc nanoparticles (ZnONPs) are particles with a size range of 1–100 nm made of zinc. Zinc oxide (ZnO) NPs are a wide band gap semiconductor with a room temperature energy gap of 3.37 eV. Its catalytic, electrical, optoelectronic, and photochemical capabilities have made it widely worthwhile ( Kumar S.S. et al., 2013 ). ZnO nanostructures are ideal for catalytic reaction processes ( Chen and Tang, 2007 ). Laser ablation, hydrothermal methods, electrochemical depositions, sol-gel method, chemical vapor deposition, thermal decomposition, combustion methods, ultrasound, microwave-assisted combustion method, two-step mechanochemical-thermal synthesis, anodization, co-precipitation, electrophoretic deposition, and precipitation processes are some methods for producing ZnO nanoparticles ( Madathil et al., 2007 ; Moghaddam et al., 2009 ; Ghorbani et al., 2015 ).
4.3. Copper nanoparticles (CuNPs)
Copper nanoparticles (CuNPs) comprise a size range of 1–100 nm of copper-based particles ( Khan et al., 2019 ). Cu and Au metal fluorescence have long been known to exist. For excitation at 488 nm, a fluorescence peak centering on the metals’ interband absorption edge has been noted. Additionally, it was noted that the fluorescence peaked at the same energy at two distinct excitation wavelengths (457.9–514.5 and 300–400 nm), and the high-energy tail somewhat grows with increased photon energy pumping. A unique, physical, top-down EEW approach has been used to create Cu nanoparticles. The EEW method involves sending a current of *1,010 A/m2 (1,010 A/m2) across a thin Cu wire, which explodes on a Cu plate for a duration of 10–6 s ( Siwach and Sen, 2008 ).
4.4. Gold nanoparticles (AuNPs)
Gold nanoparticles(AuNPs) are nanometers made of gold. They have unique physical and chemical properties and can absorb and scatter light in the visible and near-infrared range ( Rad et al., 2011 ; Compostella et al., 2017 ).
Scientists around the turn of the 20th century discovered anisotropic AuNPs. Zsigmond ( Li et al., 2014 ) said that gold particles “are not always spherical when their size is 40 nm or lower” in his book, released in 1909. Additionally, he found anisotropic gold particles of various colors. Zsigmondy won the Nobel Prize in 1925 for “his demonstration of the heterogeneous character of colloidal solutions and the methods he utilized” and for developing the ultramicroscope, which allowed him to see the forms of Au particles. He noticed that gold frequently crystallized into a six-sided leaf shape ( Li et al., 2014 ).
AuNPs are the topic of extensive investigation due to their optical, electrical, and molecular-recognition capabilities, with numerous prospective or promised uses in a wide range of fields, including electron microscopy, electronics, nanotechnology, materials science, and biomedicine ( Rad et al., 2011 ).
4.5. Aluminum nanoparticles (AlNPs)
Aluminum nanoparticles (AlNPs) are nanoparticles made of aluminum. Aluminum nanoparticles’ strong reactivity makes them promising for application in high-energy compositions, hydrogen generation in water processes, and the synthesis of alumina 2D and 3D structures ( Lerner et al., 2016 ).
4.6. Iron nanoparticles (FeNPs)
Iron nanoparticles(FeNPs) are particles with a size range of 1−100 nanometers ( Khan et al., 2019 ) made of iron. FeNPs have several potential applications, including their use as catalysts, drug delivery systems, sensors, and energy storage and conversion. They have also been investigated for use in photovoltaic and solar cells and water purification and environmental remediation. FeNPs can also be used in magnetic resonance imaging (MRI) as contrast agents to improve the visibility of tissues and organs. They can also be used in magnetic recording media, such as hard disk drives ( Zhuang and Gentry, 2011 ; Jamkhande et al., 2019 ).
As with any NPs, there are potential health and safety concerns associated with using FeNPs, e.g., FeNPs are used to deliver drugs to specific locations within the body, such as cancer cells and used in MRI, and used to remove contaminants from water ( Farrell et al., 2003 ; Zhuang and Gentry, 2011 ). Tables 1 , ,2 2 show the characteristics of metal-based nanoparticles and the techniques to study their characteristics, respectively.
Characteristics of metal based nanoparticles.
Different analytical techniques and their purposes in studying nanoparticles.
5. Approaches for the synthesis of metal NPs
There are mainly three types of approaches for the synthesis of NPs: the physical, chemical, and biological approaches. The physical approach is also called the top-down approach, while chemical and biological approaches are collectively called the bottom-up approach. The biological approach is also named green systems of NPs. All these approaches are further sub-categorized into various types based upon their method adopted. Figure 1 illustrates each approach’s reported methods for synthesizing NPs.

Approaches of NPs synthesis.
5.1. Top down/physical approach
Bulk materials are fragmented in top-down methods to create nano-structured materials ( Figure 2 ). They are additionally known as physical approaches ( Baig et al., 2021 ). The following techniques can achieve a top-down approach;

Difference between top-down and bottom-up approaches.
5.1.1. Mechanical milling
The mechanical milling process uses balls inside containers and may be carried out in various mills, typically planetary and shaker mills, which is an impact process with high energy ( Gorrasi and Sorrentino, 2015 ). Mechanical milling is a practical approach for creating materials at the nanoscale from bulk materials. Aluminum alloys that have been strengthened by oxide and carbide, spray coatings that are resistant to wear, nanoalloys based on aluminum, nickel, magnesium, and copper, and a variety of other nanocomposite materials may all be created mechanically. A unique class of nanoparticles known as ball-milled carbon nanomaterials has the potential to meet the needs for energy storage, energy conversion, and environmental remediation ( Yadav et al., 2012 ; Lyu et al., 2017 ).
5.1.2. Electrospinning
Typically, it is used to create nanofibers from various materials, most often polymers ( Ostermann et al., 2011 ). A technique for creating fibers called electrospinning draws charged threads from polymer melts or solutions up to fiber sizes of a few hundred nanometers ( Chronakis, 2010 ). Coaxial electrospinning was a significant advancement in the field of electrospinning. The spinneret in coaxial electrospinning is made up of two coaxial capillaries. Core-shell nanoarchitectures may be created in these capillaries using two viscous liquids, a viscous liquid as the shell and a non-viscous liquid as the core ( Du et al., 2012 ). Core-shell and hollow polymer, inorganic, organic, and hybrid materials have all been developed using this technique ( Kumar R. et al., 2013 ).
5.1.3. Laser ablation
A microfeature can be made by employing a laser beam to vaporize a single material ( Tran and Wen, 2014 ). Laser ablation synthesis produces nanoparticles by striking the target material with an intense laser beam. Due to the high intensity of the laser irradiation used in the laser ablation process, the source material or precursor vaporizes, causing the production of nanoparticles ( Amendola and Meneghetti, 2009 ). Laser ablation is an environmentally friendly for producing noble metal nanoparticles ( Baig et al., 2021 ). This method may be used to create a wide variety of nanomaterials, including metal nanoparticles, carbon nanomaterials, oxide composites, and ceramics ( Su and Chang, 2018 ; Baig et al., 2021 ).
5.1.4. Sputtering
Microparticles of a solid material are expelled off its surface during the phenomenon known as sputtering, which occurs when the solid substance is assaulted by intense plasma or gas particles ( Behrisch, 1981 ). According to the incident gaseous ion energy, energetic gaseous ions used in the sputtering deposition process physically expel tiny atom clusters off the target surface ( Muñoz-García et al., 2009 ). The sputtering method is intriguing because it is more affordable than electron-beam lithography, and the composition of the sputtered nanomaterials is similar to the target material with fewer contaminants ( Baig et al., 2021 ).
5.1.5. Electron explosion
In this technique, a thin metal wire is subjected to a high current pulse that causes an explosion, evaporation, and ionization. The metal becomes vaporized and ionized, expands, and cools by reacting with the nearby gas or liquid medium. The condensed vapor finally forms the nanoparticles ( Joh et al., 2013 ). Electron explosion method because it produces plasma from the electrical explosion of a metallic wire, which may produce nanoparticles from a Pt solution without using a reducing agent ( Joh et al., 2013 ).
5.1.6. Sonication
The most crucial step in the creation of nanofluids is sonication. After the mixture has been magnetically stirred in a magnetic stirrer, sonication is performed in an ultrasonication path, ultrasonic vibrator, and mechanical homogenizer. Sonicators have become the industry standard for Probe sonication and are noticeably more powerful and effective when compared to ultrasonic cleaner baths for nanoparticle applications. Probe sonication is highly effective for processing nanomaterials (carbon nanotubes, graphene, inks, metal oxides, etc.) ( Zheng et al., 2010 ).
5.1.7. Pulsed wire discharge method
This is the most used method for creating metal nanoparticles. A pulsating current causes a metal wire to evaporate, producing a vapor that is subsequently cooled by an ambient gas to form nanoparticles. This plan may quickly produce large amounts of energy ( Patil et al., 2021 ).
5.1.8. Arc discharge method
Two graphite rods are adjusted in a chamber with a constant helium pressure during the Arc Discharge procedure. It is crucial to fill the chamber with helium because oxygen or moisture prevents the synthesis of fullerenes. Arc discharge between the ends of the graphite rods drives the vaporization of carbon rods. Achieving new types of nanoparticles depends significantly on the circumstances in which arc discharge occurs. The creation of several nanostructured materials may be accomplished with this technique ( Berkmans et al., 2014 ). It is well-recognized for creating carbon-based materials such as fullerenes, carbon nanohorns (CNHs), carbon nanotubes ( Shi et al., 2000 ), few-layer graphene, and amorphous spherical carbon nanoparticles ( Kumar R. et al., 2013 ).
5.1.9. Lithography
Lithography typically uses a concentrated beam of light or electrons to create nanoparticles, a helpful technique ( Pimpin and Srituravanich, 2012 ). Masked and maskless lithography are the two primary categories of lithography. Without a mask, arbitrary nano-pattern printing is accomplished in maskless lithography. Additionally, it is affordable and easy to apply ( Brady et al., 2019 ).
5.2. Bottom-up approach
Tiny atoms and molecules are combined in bottom-up methods to create nano-structured particles ( Figure 2 ; Baig et al., 2021 ). These include chemical and biological approaches:
5.2.1. Chemical vapor deposition (CVD)
Through a chemical process involving vapor-phase precursors, a thin coating is created on the substrate surface during CVD ( Dikusar et al., 2009 ). Precursors are deemed appropriate for CVD if they exhibit sufficient volatility, high chemical purity, strong evaporation stability, cheap cost, a non-hazardous nature, and long shelf life. Additionally, its breakdown should not leave behind any contaminants. Vapor phase epitaxy, metal-organic CVD, atomic layer epitaxy, and plasma-enhanced CVD are only a few CVD variations. This method’s benefits include producing very pure nanoparticles that are stiff, homogeneous, and strong ( Ago, 2015 ). CVD is an excellent approach to creating high-quality nanomaterials ( Machac et al., 2020 ). It is also well-known for creating two-dimensional nanoparticles ( Baig et al., 2021 ).
5.2.2. Sol-gel process
A wet-chemical approach, called the sol-gel method, is widely utilized to create nanomaterials ( Das and Srivasatava, 2016 ; Baig et al., 2021 ). Metal alkoxides or metal precursors in solution are condensed, hydrolyzed, and thermally decomposed. The result is a stable solution or sol. The gel gains greater viscosity as a result of hydrolysis or condensation. The particle size may be seen by adjusting the precursor concentration, temperature, and pH levels. It may take a few days for the solvent to be removed, for Ostwald ripening to occur, and for the phase to change during the mature stage, which is necessary to enable the growth of solid mass. To create nanoparticles, the unstable chemical ingredients are separated. The generated material is environmentally friendly and has many additional benefits thanks to the sol-gel technique ( Patil et al., 2021 ). The uniform quality of the material generated, the low processing temperature, and the method’s ease in producing composites and complicated nanostructures are just a few of the sol-gel technique’s many advantages ( Parashar et al., 2020 ).
5.2.3. Co-precipitation
It is a solvent displacement technique and is a wet chemical procedure. Ethanol, acetone, hexane, and non-solvent polymers are examples of solvents. Polymer phases can be either synthetic or natural. By mixing the polymer solution, fast diffusion of the polymer-solvent into the non-solvent phase of the polymer results. Interfacial stress at two phases results in the formation of nanoparticles ( Das and Srivasatava, 2016 ). This method’s natural ability to produce high quantities of water-soluble nanoparticles through a straightforward process is one of its key benefits. This process is used to create many commercial iron oxide NP-based MRI contrast agents, including Feridex, Reservist, and Combidex ( Baig et al., 2021 ; Patil et al., 2021 ).
5.2.4. Inert gas condensation/molecular condensation
Metal NPs are produced using this method in large quantities. Making fine NPs using the inactive gas compression approach has been widespread, which creates NPs by causing a metallic source to disappear in an inert gas. At an attainable temperature, metals evaporate at a tolerable pace. Copper metal nanoparticles are created by vaporizing copper metal inside a container containing argon, helium, or neon. The atom quickly loses its energy by cooling the vaporized atom with an inert gas after it boils out. Liquid nitrogen is used to cool the gases, forming nanoparticles in the range of 2–100 nm ( Pérez-Tijerina et al., 2008 ; Patil et al., 2021 ).
5.2.5. Hydrothermal
In this method, for the production of nanoparticles, hydrothermal synthesis uses a wide temperature range from ambient temperature to extremely high temperatures. Comparing this strategy to physical and biological ones offers several benefits. At higher temperature ranges, the nanomaterials produced by hydrothermal synthesis could become unstable ( Banerjee et al., 2008 ; Patil et al., 2021 ).
5.2.6. Green/biological synthesis
The synthesis of diverse metal nanoparticles utilizing bioactive agents, including plant materials, microbes, and various biowastes like vegetable waste, fruit peel waste, eggshell, agricultural waste, algae, and so on, is known as “green” or “biological” nanoparticle synthesis ( Kumari et al., 2021 ). Developing dependable, sustainable green synthesis technologies is necessary to prevent the formation of undesirable or dangerous byproducts ( Figure 3 ). The green synthesis of nanoparticles also has several advantages, including being straightforward, affordable, producing NPs with high stability, requiring little time, producing non-toxic byproducts, and being readily scaled up for large-scale synthesis ( Malhotra and Alghuthaymi, 2022 ).

Schematic diagram for biosynthesis of NPs.
5.2.6.1. Biological synthesis using microorganisms
Microbes use metal capture, enzymatic reduction, and capping to create nanoparticles. Before being converted to nanoparticles by enzymes, metal ions are initially trapped on the surface or interior of microbial cells ( Ghosh et al., 2021 ). Use of microorganisms (especially marine microbes) for synthesis of metalic NPs is environmental friendly, fast and economical ( Patil and Kim, 2018 ). Several microorganisms are used in the synthesis of metal NPs, including:
Biosynthesis of NPs by bacteria: A possible biofactory for producing gold, silver, and cadmium sulfide nanoparticles is thought to be bacterial cells. It is known that bacteria may produce inorganic compounds either inside or outside of their cells ( Hulkoti and Taranath, 2014 ). Desulforibrio caledoiensis ( Qi et al., 2013 ), Enterococcu s sp. ( Rajeshkumar et al., 2014 ), Escherichia coli VM1 ( Maharani et al., 2016 ), and Ochrobactrum anhtropi ( Thomas et al., 2014 ) based metal NPs are reported previously for their potential photocatalytic properties ( Qi et al., 2013 ), antimicrobial activity ( Rajeshkumar et al., 2014 ), and anticancer activity ( Maharani et al., 2016 ).
Extracellular synthesis of NPs by bacteria: The microorganisms’ extracellular reductase enzymes shrink the silver ions to the nanoscale range. According to protein analysis of microorganisms, the NADH-dependent reductase enzyme carries out the bio-reduction of silver ions to AgNPs. The electrons for the reductase enzyme come from NADH, which is subsequently converted to NAD+. The enzyme is also oxidized simultaneously when silver ions are reduced to nanosilver. It has been noted that bio-reduction can occasionally be caused by nitrate-dependent reductase. The decline occurs within a few minutes in the quick extracellular creation of nanoparticles ( Mathew et al., 2010 ). At pH 7, the bacterium R. capsulata produced gold nanoparticles with sizes ranging from 10−20 nm. Numerous nanoplates and spherical gold nanoparticles were produced when the pH was changed to four ( Sriram et al., 2012 ). By adjusting the pH, the gold nanoparticles’ form may be changed. Gold nanoparticle shape was controlled by regulating the proton content at various pH levels. The bacteria R. capsulata ’s release cofactor NADH and NADH-dependent enzymes may cause the bioreduction of Au (3+) to Au (0) and the generation of gold nanoparticles. By using NADH-dependent reductase as an electron carrier, it is possible to start the reduction of gold ions ( Sriram et al., 2012 ).
Intracellular synthesis of NPs by bacteria: Three processes are involved in the intracellular creation of NPs: trapping, bioreduction, and capping. The cell walls of microorganisms and ions charge contribute significantly to creating NPs in the intracellular route. This entails specific ion transit in the presence of enzymes, coenzymes, and other molecules in the microbial cell. Microbes have a range of polysaccharides and proteins in their cell walls, which function as active sites for the binding of metal ions ( Slavin et al., 2017 ). Not all bacteria can produce metal and metal oxide nanoparticles. The only ions that pose a significant hazard to microorganisms are heavy metal ions, which, in response to a threat, cause the germs to react by grabbing or trapping the ions on the cell wall via electrostatic interactions. This occurs because a metal ion is drawn to the cell wall’s carboxylate groups, including cysteine and polypeptides, and certain enzymes with a negative charge ( Zhang et al., 2011 ).
Additionally, the electron transfers from NADH via NADH-dependent educates, which serves as an electron carrier and is located inside the plasma membrane, causing the trapped ions to be reduced into the elemental atom. The nuclei eventually develop into NPs and build up in the cytoplasm or the pre-plasmic space. On the other hand, the stability of NPs is provided by proteins, peptides, and amino acids found inside cells, including cysteine, tyrosine, and tryptophan ( Mohd Yusof et al., 2019 ).
Biosynthesis of NPs by fungi: Because monodisperse nanoparticles with distinct dimensions, various chemical compositions, and sizes may be produced, the biosynthesis of nanoparticles utilizing fungus is frequently employed. Due to the existence of several enzymes in their cells and the ease of handling, fungi are thought to be great candidates for producing metal and metal sulfide nanoparticles ( Mohanpuria et al., 2008 ).
The nanoparticles were created on the surface of the mycelia. After analyzing the results and noting the solution, it was determined that the Ag + ions are initially trapped on the surface of the fungal cells by an electrostatic interaction between gold ions and negatively charged carboxylate groups, which is facilitated by enzymes that are present in the mycelia’s cell wall. Later, the enzymes in the cell wall reduce the silver ions, causing the development of silver nuclei. These nuclei then increase as more Ag ions are reduced and accumulate on them.
The TEM data demonstrate the presence of some silver nanoparticles both on and inside the cytoplasmic membrane. The findings concluded that the Ag ions that permeate through the cell wall were decreased by enzymes found inside the cytoplasm and on the cytoplasmic membrane. Also possible is the diffusion of some silver nanoparticles over the cell wall and eventual cytoplasmic entrapment ( Mukherjee et al., 2001 ; Hulkoti and Taranath, 2014 ).
It was observed that the culture’s age does not affect the shape of the synthesized gold nanoparticles. However, the number of particles decreased when older cells were used. The different pH levels produce a variety of shapes of gold nanoparticles, indicating that pH plays a vital role in determining the shape. The incubation temperature also played an essential role in the accumulation of the gold nanoparticles. It was observed that the particle growth rate was faster at increased temperature levels ( Mukherjee et al., 2001 ; Ahmad et al., 2003 ). The form of the produced gold nanoparticles was shown to be unaffected by the age of the culture. However, when older cells were utilized, the particle count fell. The fact that gold nanoparticles take on various forms at different pH levels suggests that the pH is crucial in determining the shape. The incubation temperature significantly influenced the accumulation of the gold nanoparticles. It was found that higher temperatures caused the particle development rate to accelerate ( Mukherjee et al., 2001 ; Ahmad et al., 2003 ). Verticillium luteoalbum is reported to synthesize gold nanoparticles of 20–40 nm in size ( Erasmus et al., 2014 ). Aspergillus terreus and Penicillium brevicompactum KCCM 60390 based metal NPs are reported for their antimicrobial ( Li G. et al., 2011 ) and cytotoxic activities ( Mishra et al., 2011 ), respectively.
Biosynthesis of NPs using actinomycetes: Actinomycetes have been categorized as prokaryotes since they share significant traits with fungi. They are sometimes referred to as ray fungi ( Mathew et al., 2010 ). Making NPs from actinomycetes is the same as that of fungi ( Sowani et al., 2016 ). Thermomonospora sp., a new species of extremophilic actinomycete, was discovered to produce extracellular, monodispersed, spherical gold nanoparticles with an average size of 8 nm ( Narayanan and Sakthivel, 2010 ). Metal NPs synthesized by Rhodococcus sp. ( Ahmad et al., 2003 ) and Streptomyces sp. Al-Dhabi-87 ( Al-Dhabi et al., 2018 ) are reported for their antimicrobial activities.
Biosynthesis of NPs using algae: Algae have a high concentration of polymeric molecules, and by reducing them, they may hyper-accumulate heavy metal ions and transform them into malleable forms. Algal extracts typically contain pigments, carbohydrates, proteins, minerals, polyunsaturated fatty acids, and other bioactive compounds like antioxidants that are used as stabilizing/capping and reducing agents ( Khanna et al., 2019 ). NPs also have a faster rate of photosynthesis than their biosynthetic counterparts. Live or dead algae are used as model organisms for the environmentally friendly manufacturing process of bio-nanomaterials, such as metallic NPs ( Hasan, 2015 ). Ag and Au are the most extensively researched noble metals to synthesized NPs by algae either intracellularly or extracellularly ( Dahoumane et al., 2017 ). Chlorella vulgaris ( Luangpipat et al., 2011 ), Chlorella pyrenoidosa ( Eroglu et al., 2013 ), Nanochloropsis oculata ( Xia et al., 2013 ), Scenedesmus sp. IMMTCC-25 ( Jena et al., 2014 ) based metal NPs are reported for their potential catalytic ( Luangpipat et al., 2011 ; Eroglu et al., 2013 ) and, antimicrobial ( Eroglu et al., 2013 ; Jena et al., 2014 ) activities along with their use in Li-Ion batteries ( Xia et al., 2013 ).
Intracellular synthesis of NPs using algae: In order to create intracellular NPs, algal biomass must first be gathered and thoroughly cleaned with distilled water. After that, the biomass (living algae) is treated with metallic solutions like AgNO3. The combination is then incubated at a specified pH and a specific temperature for a predetermined time. Finally, it is centrifuged and sonicated to produce the extracted stable NPs ( Uzair et al., 2020 ).
Extracellular synthesis of NPs using algae: Algal biomass is first collected and cleaned with distilled water before being used to synthesize NPs extracellularly ( Uzair et al., 2020 ). The following three techniques are frequently utilized for the subsequent procedure:
(i) A particular amount of time is spent drying the algal biomass (dead algae), after which the dried powder is treated with distilled water and filtered.
(ii) The algal biomass is sonicated with distilled water to get a cell-free extract.
(iii) The resultant product is filtered after the algal biomass has been rinsed with distilled water and incubated for a few hours (8–16 h).
5.2.6.2. Biological synthesis using plant extracts
The substance or active ingredient of the desired quality extracted from plant tissue by treatment for a particular purpose is a plant extract ( Jadoun et al., 2021 ). Plant extracts are combined with a metal salt solution at room temperature to create nanoparticles. Within minutes, the response is finished. This method has been used to create nanoparticles of silver, gold, and many other metals ( Li X. et al., 2011 ). Nanoparticles are biosynthesized using a variety of plants. It is known that the kind of plant extract, its concentration, the concentration of the metal salt, the pH, temperature, and the length of contact time all have an impact on how quickly nanoparticles are produced as well as their number and other properties ( Mittal and Chisti, 2013 ). A leaf extract from Polyalthia longifolia was used to create silver nanoparticles, the average particle size was around 58 nm ( Kumar and Yadav, 2009 ; Kumar et al., 2016 ).
Acacia auriculiformis ( Saini et al., 2016 ), Anisomeles indica ( Govindarajan et al., 2016 ), Azadirachta indica ( Velusamy et al., 2015 ), Bergenia ciliate ( Phull et al., 2016 ), Clitoria ternatea , Solanum nigrum ( Krithiga et al., 2013 ), Coffea arabica ( Dhand et al., 2016 ), Coleus forskohlii ( Naraginti et al., 2016 ), Curculigo orchioides ( Kayalvizhi et al., 2016 ), Digitaria radicosa ( Kalaiyarasu et al., 2016 ), Dioscorea alata ( Pugazhendhi et al., 2016 ), Diospyros paniculata ( Rao et al., 2016 ), Elephantopus scaber ( Kharat and Mendhulkar, 2016 ), Emblica officinalis ( Ramesh et al., 2015 ), Euphorbia antiquorum L. ( Rajkuberan et al., 2017 ), Ficus benghalensis ( Nayak et al., 2016 ), Lantana camara ( Ajitha et al., 2015 ), Cinnamomum zeylanicum ( Soni and Sonam, 2014 ), and Parkia roxburghii ( Paul et al., 2016 ) are the few examples of plants which are reported for the green synthesis of metal NPs (i.e., AgNPs). These were evaluated for their antifilaria activity ( Saini et al., 2016 ), mosquitocidal activity ( Govindarajan et al., 2016 ), antibacterial activity ( Velusamy et al., 2015 ), catalytic activity ( Edison et al., 2016 ), antioxidant activity ( Phull et al., 2016 ), and Cytotoxicity ( Patil et al., 2017 ).
5.2.6.3. Biological synthesis using biomimetic
“Biomimetic synthesis” typically refers to chemical processes that resemble biological synthesis carried out by living things ( Dahoumane et al., 2017 ). In the biomimetic approach, proteins, enzymes, cells, viruses, pollen, and waste biomass are used to synthesize NPs. Two categories are used to classify biomimetic synthesis:
Functional biomimetic synthesis uses various materials and approaches to emulate particular characteristics of natural materials, structures, and systems ( Zan and Wu, 2016 ).
Process biomimetic synthesis is a technique that aims to create different desirable nanomaterials/structures by imitating the synthesis pathways, processes, or procedures of natural chemicals and materials/structures. For instance, several distinctive nano-superstructures (such as satellite structures, dendrimer-like structures, pyramids, cubes, 2D nanoparticle arrays, 3D AuNP tubes, etc.) have been put together in vitro by simulating the protein manufacturing process ( Zan and Wu, 2016 ).
6. Applications of NPs
6.1. applications of nps in environment industry.
Due to their tiny size and distinctive physical and chemical characteristics, NPs appeal to various environmental applications. The properties of nanoparticals and their advantages are illustrated in Figure 4 . The following are some possible NP uses in the environment.

Properties of nanoparticals and their advantages.
6.1.1. Bioremediation
Nanoparticles (NPs) can remove environmental pollutants, such as heavy metals from water or organic contaminants from soil ( Zhuang and Gentry, 2011 ). For example, silver nanoparticles (AgNPs) effectively degrade certain pollutants, such as organic dyes and compounds found in wastewater. Several nanomaterials have been considered for remediation purposes, such as nanoscale zeolites, metal oxides, and carbon nanotubes and fibers ( Zhuang and Gentry, 2011 ). Nanoscale particles used in remediation can access areas that larger particles cannot. They can be coated to facilitate transport and prevent reaction with surrounding soil matrices before reacting with contaminants. One widely used nanomaterial for remediation is Nanoscale zerovalent iron (nZVI). It has been used at several hazardous waste sites to clean up chlorinated solvents that have contaminated groundwater ( Elliott et al., 2013 ). Removing heavy metals such as mercury, lead, thallium, cadmium, and arsenic from natural water has attracted considerable attention because of their adverse effects on environmental and human health. Superparamagnetic iron oxide NPs are an effective sorbent material for this toxic soft material. So, no measurements of engineered NPs in the environment have been available due to the absence of analytical methods able to quantify the trace concentration of NPs ( Elliott et al., 2013 ).
6.1.2. Sensors in environment
Nanotechnology/NPs are already being used to improve water quality and assist in environmental clean-up activities ( Pradeep, 2009 ). Their potential use as environmental sensors to monitor pollutants is also becoming viable NPs can be used as sensors to detect the presence of certain compounds in the environment, such as heavy metals or pollutants. The nano-sensors small size and wide detection range provide great flexibility in practical applications. It has been reported that nanoscale sensors can be used to detect microbial pathogens and biological compounds, such as toxins, in aqueous environments ( Yadav et al., 2010 ). NPS can be designed to selectively bind to specific types of pollutants, allowing them to be detected at low concentrations. For example, gold nanoparticles (AuNPs) have been used as sensors for the detection of mercury in water ( Theron et al., 2010 ).
6.1.3. Catalysts in environment
Nanoparticles (NPs) are used as catalysts in chemical reactions, such as in the production of biofuels or environmental remediation processes, and to catalyze biomass conversion into fuels, such as ethanol or biodiesel. For example, platinum nanoparticles (PtNPs) have been explored for use in the production of biofuels due to their ability to catalyze the conversion of biomass into fuels ( Lam and Luong, 2014 ). PtNPs also showed promising sensing properties; for example, Using Pt NPs, the Hg ions were quantified in the range of 50–500 nM in MilliQ, tap, and groundwater samples, and the limit of quantifications for Hg ions were 16.9, 26, and 47.3 nM. The biogenic PtNPs-based probe proved to be applicable for detecting and quantifying Hg ions ( Kora and Rastogi, 2018 ).
Overall, NPs have significant potential for use in the environment and are being actively researched for a variety of applications.
6.2. Applications of NPs in medicine industry
Nanoparticles (NPs) have unique physical and chemical properties due to their small size, making them attractive for use in various applications, including the medicine industry. Some potential applications of NPs in medicine include:
6.2.1. Drug delivery
Technological interest has been given to AuNPs due to their unique optical properties, ease of synthesis, and chemical stability. The particles can be used in biomedical applications such as cancer treatment ( Sun et al., 2014 ), biological imaging ( Abdulle and Chow, 2019 ), chemical sensing, and drug delivery. Sun et al. (2014) mentioned in detail about two different methods of controlled release of drugs associated with NPs, which were (1) sustained (i.e., diffusion-controlled and erosion-controlled) and (2) stimuli-responsive (i.e., pH-sensitive, enzyme-sensitive, thermoresponsive, and photosensitive). Figure 5 illustrates that how NPs acts as targeted delivery of medicines to treat cancer cells ( Figure 5A ) and therapeutic gene delivery to synthesis proteins of interests in targeted cells ( Figure 5B ). NPs can deliver drugs to specific body areas, allowing for more targeted and effective treatment ( Siddique and Chow, 2020 ). For example AgNPs have been explored for use in drug delivery due to their stability and ability to accumulate in certain types of cancerous tumors ( Siddique and Chow, 2020 ). ZnONPs have also been explored for drug delivery due to their ability to selectively target cancer cells ( Anjum et al., 2021 ). CuNPs have been shown to have antimicrobial properties and are being explored for drug delivery to treat bacterial infections ( Yuan et al., 2018 ). AuNPs have unique optical, electrical, and catalytic properties and are being explored for drug delivery due to their ability to accumulate in certain cancerous tumors. Silver NPs (AgNPs) have been incorporated into wound dressings, bone cement, and implants ( Schröfel et al., 2014 ).

Application of nanoparticles as; targated drug delivery (A) , and therapeutic protein generation in targated cells (B) .
6.2.2. Diagnostics
Nanoparticles (NPs) can be used as imaging agents to help visualize specific body areas. For example, iron oxide nanoparticles (Fe 3 O 4 NPs) have been used as magnetic resonance imaging (MRI) contrast agents to help visualize tissues and organs ( Nguyen et al., 2013 ). AuNPs have unique optical, electrical, and catalytic properties and are being explored for diagnostics due to their ability to accumulate in certain cancerous tumors ( Siddique and Chow, 2020 ).
6.2.3. Tissue engineering
Nanoparticles (NPs) can help stimulate the growth and repair of tissues and organs. For example, titanium dioxide nanoparticles (TiO2 NPs) have been explored for tissue engineering due to their ability to stimulate the growth of bone cells ( Kim et al., 2014 ).
6.2.4. Antimicrobials
Some NPs, such as silver nanoparticles (AgNPs) and copper nanoparticles (CuNPs), have strong antimicrobial properties and are being explored for use in a variety of medical products, such as wound dressings and medical devices ( Hoseinzadeh et al., 2017 ).
Overall, NPs have significant potential for use in the medical industry and are being actively researched for various applications. However, it is essential to carefully consider the potential risks and benefits of using NPs in medicine and ensure their safe and responsible use.
6.3. Applications of NPs in agriculture industry
There are several ways in which nanoparticles (NPs) have the potential to alter the agricultural sector. NPs may be used in agriculture for a variety of reasons, including:
6.3.1. Pesticides and herbicides
Nanoparticles (NPs) can be used to deliver pesticides and herbicides in a targeted manner, reducing the number of chemicals needed and minimizing the potential for environmental contamination ( Khan et al., 2019 ). AgNPs and CuNPs have antimicrobial properties, making them potentially useful for controlling pests and diseases in crops. They can also be used as delivery systems for active ingredients, allowing for more targeted application and reducing the potential for environmental contamination ( Hoseinzadeh et al., 2017 ; Dangi and Verma, 2021 ).
It is important to note that using metal NPs in pesticides and herbicides is still in the early stages of development. More research is needed to understand their potential impacts on human health and the environment ( Dangi and Verma, 2021 ).
6.3.2. Fertilizers and plant growth
Nano fertilizers offer an opportunity for efficiently improving plant mineral nutrition. Some studies have shown that nanomaterials can be more effective than conventional fertilizers, with a controlled release of nutrients increasing the efficiency of plant uptake and potentially reducing adverse environmental outcomes associated with the loss of nutrients in the broader environment. However, other studies have found that nanomaterial has the same or even less effective effectiveness than conventional fertilizers. NPs used to deliver fertilizers to plants more efficiently, reducing the amount of fertilizer needed, and reducing the risk of nutrient runoff ( Kopittke et al., 2019 ).
Ag ( Jaskulski et al., 2022 ), Zn ( Song and Kim, 2020 ), Cu, Au, Al, and Fe ( Kopittke et al., 2019 ) based NPs have been shown to have fertilizing properties and plant growth-promoting properties, and may help provide essential nutrients to plants and improve plant growth and yield. It is important to note that the use of NPs in fertilizers is still in the early stages of development. More research is needed to understand their potential impacts on human health and the environment.
6.3.3. Food safety
Nanoparticles (NPs) can detect and eliminate pathogens in food products, improving food safety, and reducing the risk of foodborne illness ( Zhuang and Gentry, 2011 ).
6.3.4. Water purification
Nanoparticles (NPs) can purify irrigation water, reducing the risk of crop contamination and improving crop yield ( Zhuang and Gentry, 2011 ). Using NPs in agriculture can improve crop yields, reduce agriculture’s environmental impact, and improve food products’ safety and quality.
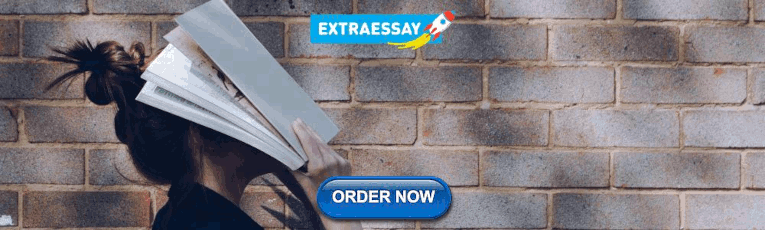
6.4. Applications of NPs in food industry
Numerous applications for nanoparticles (NPs) in the food sector are possible, including:
6.4.1. Food processing and food preservation/food packaging
Nanoparticles (NPs) can be used to improve the efficiency and performance of food processing operations, such as grinding, mixing, and drying, e.g., AgNPs have been used as a natural antimicrobial agent in food processing operations, helping to prevent the growth of bacteria and other microorganisms ( Dangi and Verma, 2021 ) and also NPs are used to enhance the performance of materials used in food packaging, making them more resistant to pollutants like moisture and gases.
6.4.2. Food fortification
Nanoparticles (NPs) can deliver essential nutrients to food products, such as vitamins and minerals, more efficiently and effectively. e.g., Fe 2 O 3 , and CuNPs have been used to fortify food products with iron, and Cu is an essential nutrient necessary for the metabolism of iron and other nutrients. Iron is an essential nutrient often lacking in many people’s diets, particularly in developing countries ( Kopittke et al., 2019 ).
6.4.3. Sensors
Nanoparticles (NPs) used to improve the sensitivity and specificity of food sensors, allowing them to detect a broader range of substances or signals ( Yadav et al., 2010 ).
Overall, using NPs in the food industry can improve the performance, safety, and nutritional value of a wide range of food products and processes.
6.5. Applications of NPs in electronics industry and automotive industry
In many aspects, nanoparticles (NPs) can transform the electronics sector. NPs may be used in a variety of electrical applications, such as:
6.5.1. Display technologies/storage devices
Nanoparticles (NPs) can be used to improve the performance of displays ( Park and Choi, 2019 ; Bahadur et al., 2021 ; Triana et al., 2022 ), such as LCD and OLED displays, by enhancing the brightness, color, and contrast of the image, such as silver NPs and gold NPs, have been explored for use in LCD and OLED displays as a means of improving the conductivity of the display ( Gwynne, 2020 ). NPs improve the performance and durability of energy storage devices, such as batteries and supercapacitors, by increasing energy density and charging speed. Zinc oxide nanoparticles (ZnO NPs) have the potential to be used in energy storage devices, such as batteries and supercapacitors, due to their ability to store and release energy ( Singh et al., 2011 ).
6.5.2. Data storage
Nanoparticles (NPs) can improve the capacity and speed of data storage devices, such as hard drives and flash drives. Magnetic NPs, such as iron oxide NPs, have been explored for use in data storage devices, such as hard drives, due to their ability to store, and retrieve data using magnetism. These NPs are often composed of a magnetic metal, such as iron, cobalt, or nickel. They can be magnetized and demagnetized, allowing them to store and retrieve data ( Ahmad et al., 2021 ).
Overall, the use of NPs in electronics has the potential to improve the performance and efficiency of a wide range of electronic devices and systems.
Applications of NPs in chemical industry: The chemical industry might be entirely transformed by nanoparticles (NPs) in various ways. The following are potential uses for NPs in the chemical industry ( Salem and Fouda, 2021 ).
6.5.3. Chemical processing/catalysis
Nanoparticles (NPs) can be used as catalysts in chemical reactions, allowing them to be carried out more efficiently and at lower temperatures. Some examples of metal NPs that have been used as catalysts in the chemical industry include: PtNPs have been used as catalysts in a variety of chemical reactions, including fuel cell reactions ( Bhavani et al., 2021 ), hydrogenation reactions, and oxidation reactions ( Lara and Philippot, 2014 ), PdNPs have been used as catalysts in a variety of chemical reactions, including hydrogenation reactions and cross-coupling reactions ( Pérez-Lorenzo, 2012 ), FeNPs have been used as catalysts in a variety of chemical reactions, including hydrolysis reactions ( Jiang and Xu, 2011 ), and oxygen reduction reactions, NiNPs have been used as catalysts in a variety of chemical reactions, including hydrogenation reactions, and hydrolysis reactions ( Salem and Fouda, 2021 ).
6.5.4. Separation and purification
NPs are used to separate and purify chemicals and other substances, such as gases and liquids, by exploiting their size-based properties ( Hollamby et al., 2010 ). Several types of metal nanoparticles (NPs) have been explored for use in separation and purification processes in the chemical industry, including Fe 2 O 3 NPs have been used to separate and purify gases, liquids, and chemicals. They have also been used to remove contaminants from water ( Pradeep, 2009 ; Siddique and Chow, 2020 ). AgNPs have been used to purify water and remove contaminants ( Pradeep, 2009 ), such as bacteria and viruses. They have also been used to remove heavy metals from water and other substances ( Zhuang and Gentry, 2011 ). AuNPs have been used to purify water and remove contaminants, such as bacteria and viruses ( Siddique and Chow, 2020 ). They have also been used to separate and purify gases and liquids ( Zhuang and Gentry, 2011 ). AlNPs have been used to remove contaminants from water and other substances, such as oils and fuels. They have also been used to purify gases ( Zhuang and Gentry, 2011 ).
6.6. Applications of NPs in defense industry
Nanoparticles (NPs) can be used to improve the efficiency and performance of chemical processing operations, such as refining and synthesizing chemicals ( Schröfel et al., 2014 ). Nanoparticles (NPs) have the potential to be used in the defense industry in several ways, including:
6.6.1. Sensors
Nanoparticles (NPs) can improve the sensitivity and specificity of sensors used in defense systems, such as sensors for detecting chemical, biological, or radiological threats ( Zheng et al., 2010 ).
6.6.2. Protective coatings
Nanoparticles (NPs) can improve the performance and durability of protective coatings applied to defense equipment, such as coatings resistant to chemical or biological agents. For example, metal NPs can improve the mechanical properties and durability of the coating, making it more resistant to wear and corrosion. For example, adding Al or Zn based NPs to a polymer coating can improve its corrosion resistance. In contrast, adding Ni or Cr-based NPs can improve their wear resistance ( Rangel-Olivares et al., 2021 ).
6.6.3. Weapons
Nanoparticles (NPs) are used as weapons against viruses, bacteria, etc, ( Ye et al., 2020 ) and as well as in the development of armor and protective materials. There have been some reports of the potential use of NPs in military and defense applications, such as in the development of armor and protective materials. For example, adding nanoparticles, such as ceramic or metal NPs, to polymers or other materials can improve their mechanical properties and make them more resistant to damage. In addition, there have been reports of the use of NPs in developing sensors and detection systems for defense purposes.
6.6.4. Manufacturing
Nanoparticles (NPs) can improve the performance and durability of materials used in defense equipment, such as armor or structural materials. Metal NPs can be used in materials by adding them as a filler or reinforcement in polymers. For example, the addition of metal NPs such as aluminum (Al), copper (Cu), or nickel (Ni) to polymers can improve the mechanical properties, thermal stability, and electrical conductivity of the resulting composite material ( Khan et al., 2019 ).
Metal NPs can also make functional materials, such as catalysts and sensors. For example, metal NPs, such as gold (Au), and platinum (Pt), can be used as catalysts in various chemical reactions due to their high surface area and ability to adsorb reactants ( Zheng et al., 2010 ).
6.6.5. Energy storage
Nanoparticles (NPs) can improve the performance and efficiency of energy storage systems used in defense systems, such as batteries or fuel cells ( Morsi et al., 2022 ). In batteries, nanoparticles can be used as a cathode material to increase the battery’s energy density, rate capability, and cycling stability. For example, lithium cobalt oxide (LiCoO 2 ) nanoparticles have been used as cathode materials in lithium-ion batteries due to their high capacity and good rate performance. In addition, nanoparticles of transition metal oxides, such as iron oxide (Fe 2 O 3 ), and manganese oxide (MnO 2 ), have been used as cathode materials in rechargeable lithium batteries due to their high capacity and good rate performance. In supercapacitors, nanoparticles can be used as the active material in the electrodes to increase the specific surface area, leading to an increase in the device’s capacitance ( Morsi et al., 2022 ). Using NPs in the defense industry can improve defense systems’ performance, efficiency, and safety.
7. Future perspectives
Metal nanoparticles (NPs) have many potential applications in various fields, including electronics, energy storage, catalysis, and medicine. However, there are also several challenges and potential future directions for developing and using metal NPs.
One major challenge is synthesizing and processing metal NPs with precise size and shape control. Many methods for synthesizing metal NPs involve high temperatures and harsh chemical conditions, which can be challenging to scale up for large-scale production. In addition, the size and shape of metal NPs can significantly impact their properties and potential applications, so it is essential to synthesize NPs with precise size and shape control.
Another challenge is the environmental impact of metal NPs. Some metal NPs, such as silver NPs, can be toxic to aquatic life and may have other environmental impacts. There is a need for more research on the environmental effects of metal NPs and the development of more environmentally friendly (Green) synthesis and processing methods.
In terms of future directions, one promising area is the use of metal NPs for energy storage, conversion, and protection of the environment. For example, metal NPs could be used to improve batteries’ performance or develop more efficient solar cells. In addition, metal NPs could be used in catalysis to improve the efficiency of chemical reactions. There is also ongoing research on metal NPs in medicine, including drug delivery and cancer therapy.
Author contributions
KAA: conceptualization, methodology, validation, formal analysis, investigation, writing – original draft, writing – review and editing, and visualization.
Acknowledgments
The author thanks Prof. Dr. Mona M. Sobhy, Department of Reproductive Diseases, Animal Reproduction Research Institute, ARC, Giza, Egypt, and Dr. Omar Hewedy, University of Guelph, Canada, for the critical reading of the manuscript.
Conflict of interest
The author declares that the research was conducted in the absence of any commercial or financial relationships that could be construed as a potential conflict of interest.
Publisher’s note
All claims expressed in this article are solely those of the authors and do not necessarily represent those of their affiliated organizations, or those of the publisher, the editors and the reviewers. Any product that may be evaluated in this article, or claim that may be made by its manufacturer, is not guaranteed or endorsed by the publisher.
- Abdulle A., Chow J. C. (2019). Contrast enhancement for portal imaging in nanoparticle-enhanced radiotherapy: A Monte Carlo phantom evaluation using flattening-filter-free photon beams. Nanomaterials 9 : 920 . 10.3390/nano9070920 [ PMC free article ] [ PubMed ] [ CrossRef ] [ Google Scholar ]
- Ago H. (2015). “ CVD growth of high-quality single-layer graphene ,” in Frontiers of Graphene and Carbon Nanotubes , Ed. Matsumoto K. (Berlin: Springer; ), 3–20. 10.1007/978-4-431-55372-4_1 [ CrossRef ] [ Google Scholar ]
- Ahmad A., Alsaad A., Al-Bataineh Q. M., Al-Akhras M.-A. H., Albataineh Z., Alizzy K. A., et al. (2021). Synthesis and characterization of ZnO NPs-doped PMMA-BDK-MR polymer-coated thin films with UV curing for optical data storage applications. Polymer Bull. 78 1189–1211. 10.1007/s00289-020-03155-x [ CrossRef ] [ Google Scholar ]
- Ahmad A., Senapati S., Khan M. I., Kumar R., Ramani R., Srinivas V., et al. (2003). Intracellular synthesis of gold nanoparticles by a novel alkalotolerant actinomycete. Rhodococcus species. Nanotechnology 14 : 824 . 10.1088/0957-4484/14/7/323 [ CrossRef ] [ Google Scholar ]
- Ahmad T., Wani I. A., Ahmed J., Al-Hartomy O. A. (2014). Effect of gold ion concentration on size and properties of gold nanoparticles in TritonX-100 based inverse microemulsions. Appl. Nanosci. 4 491–498. 10.1007/s13204-013-0224-y [ CrossRef ] [ Google Scholar ]
- Ajitha B., Reddy Y. A. K., Reddy P. S. (2015). Green synthesis and characterization of silver nanoparticles using Lantana camara leaf extract. Mater. Sci. Eng. C 49 373–381. 10.1016/j.msec.2015.01.035 [ PubMed ] [ CrossRef ] [ Google Scholar ]
- Al-Dhabi N. A., Mohammed Ghilan A.-K., Arasu M. V. (2018). Characterization of silver nanomaterials derived from marine Streptomyces sp. al-dhabi-87 and its in vitro application against multidrug resistant and extended-spectrum beta-lactamase clinical pathogens. Nanomaterials 8 : 279 . 10.3390/nano8050279 [ PMC free article ] [ PubMed ] [ CrossRef ] [ Google Scholar ]
- Amendola V., Meneghetti M. (2009). Laser ablation synthesis in solution and size manipulation of noble metal nanoparticles. Phys. Chem. Chem. Phys. 11 3805–3821. 10.1039/b900654k [ PubMed ] [ CrossRef ] [ Google Scholar ]
- Anjum S., Hashim M., Malik S. A., Khan M., Lorenzo J. M., Abbasi B. H., et al. (2021). Recent advances in zinc oxide nanoparticles (Zno nps) for cancer diagnosis, target drug delivery, and treatment. Cancers 13 : 4570 . 10.3390/cancers13184570 [ PMC free article ] [ PubMed ] [ CrossRef ] [ Google Scholar ]
- Astefanei A., Núñez O., Galceran M. T. (2015). Characterisation and determination of fullerenes: a critical review. Anal. Chim. Acta 882 1–21. [ PubMed ] [ Google Scholar ]
- Bahadur P. S., Jaiswal S., Srivastava R., Kumar A. (2021). “ Advanced application of nanotechnology in engineering ,” in Proceedings of the 2021 International Conference on Technological Advancements and Innovations (ICTAI) , (Piscataway, NJ: IEEE; ), 92–95. [ Google Scholar ]
- Baig N., Kammakakam I., Falath W. (2021). Nanomaterials: A review of synthesis methods, properties, recent progress, and challenges. Mater. Adv. 2 1821–1871. [ Google Scholar ]
- Banerjee A., Krishna R., Das B. (2008). Size controlled deposition of Cu and Si nano-clusters by an ultra-high vacuum sputtering gas aggregation technique. Appl. Phys. A 90 299–303. [ Google Scholar ]
- Bayda S., Adeel M., Tuccinardi T., Cordani M., Rizzolio F. (2019). The history of nanoscience and nanotechnology: from chemical–physical applications to nanomedicine. Molecules 25 : 112 . 10.3390/molecules25010112 [ PMC free article ] [ PubMed ] [ CrossRef ] [ Google Scholar ]
- Behrisch R. (1981). Sputtering by Particle Bombardment Springer Verlag. Berlin-Heidelberg: Springer. [ Google Scholar ]
- Berkmans A. J., Jagannatham M., Priyanka S., Haridoss P. (2014). Synthesis of branched, nano channeled, ultrafine and nano carbon tubes from PET wastes using the arc discharge method. Waste Manag. 34 2139–2145. 10.1016/j.wasman.2014.07.004 [ PubMed ] [ CrossRef ] [ Google Scholar ]
- Beyene H. D., Werkneh A. A., Bezabh H. K., Ambaye T. G. (2017). Synthesis paradigm and applications of silver nanoparticles (AgNPs), a review. Sustain. Mater. Technol. 13 18–23. [ Google Scholar ]
- Bhattacharjee S. (2016). DLS and zeta potential–what they are and what they are not? J. Control. Release 235 337–351. [ PubMed ] [ Google Scholar ]
- Bhavani K. S., Anusha T., Brahman P. K. (2021). Platinum nanoparticles decorated on graphitic carbon nitride-ZIF-67 composite support: An electrocatalyst for the oxidation of butanol in fuel cell applications. Int. J. Hydr. Energy 46 9199–9214. [ Google Scholar ]
- Biju V., Itoh T., Anas A., Sujith A., Ishikawa M. (2008). Semiconductor quantum dots and metal nanoparticles: syntheses, optical properties, and biological applications. Anal. Bioanal. Chem. 391 2469–2495. [ PubMed ] [ Google Scholar ]
- Brady B., Wang P. H., Steenhoff V., Brolo A. G. (2019). “ Nanostructuring solar cells using metallic nanoparticles ,” in Metal Nanostructures for Photonics , eds Kassab L. R. P., De Araujo C. B. (Amsterdam: Elsevier; ), 197–221. [ Google Scholar ]
- Cadene A., Durand-Vidal S., Turq P., Brendle J. (2005). Study of individual Na-montmorillonite particles size, morphology, and apparent charge. J. Colloid Interf. Sci. 285 719–730. 10.1016/j.jcis.2004.12.016 [ PubMed ] [ CrossRef ] [ Google Scholar ]
- Chen J., Zhu X. (2016). Magnetic solid phase extraction using ionic liquid-coated core–shell magnetic nanoparticles followed by high-performance liquid chromatography for determination of Rhodamine B in food samples. Food Chem. 200 10–15. 10.1016/j.foodchem.2016.01.002 [ PubMed ] [ CrossRef ] [ Google Scholar ]
- Chen J., Guo Y., Zhang X., Liu J., Gong P., Su Z., et al. (2023). Emerging nanoparticles in food: sources, application, and safety. J. Agricult. Food Chem. 71 3564–3582. [ PubMed ] [ Google Scholar ]
- Chen J., Wei S., Xie H. (2021). “ A brief introduction of carbon nanotubes: history, synthesis, and properties ,” in Proceedings of the Journal of Physics: Conference Series , (United Kingdom: IOP Publishing; ), 012184. 10.1088/1742-6596/1948/1/012184 [ CrossRef ] [ Google Scholar ]
- Chen J.-C., Tang C.-T. (2007). Preparation and application of granular ZnO/Al2O3 catalyst for the removal of hazardous trichloroethylene. J. Hazardous Mater. 142 88–96. 10.1016/j.jhazmat.2006.07.061 [ PubMed ] [ CrossRef ] [ Google Scholar ]
- Chronakis I. S. (2010). Micro-/nano-fibers by electrospinning technology: processing, properties and applications. Micromanufact. Eng. Technol. 2010 264–286. 10.1016/B978-0-8155-1545-6.00016-8 [ CrossRef ] [ Google Scholar ]
- Compostella F., Pitirollo O., Silvestri A., Polito L. (2017). Glyco-gold nanoparticles: synthesis and applications. Beilstein J. Org. Chem. 13 1008–1021. 10.3762/bjoc.13.100 [ PMC free article ] [ PubMed ] [ CrossRef ] [ Google Scholar ]
- Dahoumane S. A., Mechouet M., Wijesekera K., Filipe C. D., Sicard C., Bazylinski D. A., et al. (2017). Algae-mediated biosynthesis of inorganic nanomaterials as a promising route in nanobiotechnology–a review. Green Chem. 19 552–587. 10.1039/C6GC02346K [ CrossRef ] [ Google Scholar ]
- Dangi K., Verma A. K. (2021). Efficient & eco-friendly smart nano-pesticides: Emerging prospects for agriculture. Mater. Today Proc. 45 3819–3824. [ Google Scholar ]
- Das S., Srivasatava V. C. (2016). Synthesis and characterization of ZnO–MgO nanocomposite by co-precipitation method. Smart Sci. 4 190–195. [ Google Scholar ]
- De La Calle I., Menta M., Klein M., Séby F. (2018). Study of the presence of micro-and nanoparticles in drinks and foods by multiple analytical techniques. Food Chem. 266 133–145. 10.1016/j.foodchem.2018.05.107 [ PubMed ] [ CrossRef ] [ Google Scholar ]
- Delvallée A., Feltin N., Ducourtieux S., Trabelsi M., Hochepied J. (2015). Direct comparison of AFM and SEM measurements on the same set of nanoparticles. Measur. Sci. Technol. 26 : 085601 . [ Google Scholar ]
- Dhand V., Soumya L., Bharadwaj S., Chakra S., Bhatt D., Sreedhar B. (2016). Green synthesis of silver nanoparticles using Coffea arabica seed extract and its antibacterial activity. Mater. Sci. Eng. C 58 36–43. 10.1016/j.msec.2015.08.018 [ PubMed ] [ CrossRef ] [ Google Scholar ]
- Dikusar A., Globa P., Belevskii S., Sidel’nikova S. (2009). On limiting rate of dimensional electrodeposition at meso-and nanomaterial manufacturing by template synthesis. Surf. Eng. Appl. Electrochem. 45 171–179. [ Google Scholar ]
- Dragovic R. A., Gardiner C., Brooks A. S., Tannetta D. S., Ferguson D. J., Hole P., et al. (2011). Sizing and phenotyping of cellular vesicles using nanoparticle tracking analysis. Nanomedicine 7 780–788. [ PMC free article ] [ PubMed ] [ Google Scholar ]
- Dreaden E. C., Alkilany A. M., Huang X., Murphy C. J., El-Sayed M. A. (2012). The golden age: gold nanoparticles for biomedicine. Chem. Soc. Rev. 41 2740–2779. 10.1039/c1cs15237h [ PMC free article ] [ PubMed ] [ CrossRef ] [ Google Scholar ]
- Du P., Song L., Xiong J., Li N., Xi Z., Wang L., et al. (2012). Coaxial electrospun TiO2/ZnO core–sheath nanofibers film: Novel structure for photoanode of dye-sensitized solar cells. Electrochim. Acta 78 392–397. [ Google Scholar ]
- Edison T. N. J. I., Lee Y. R., Sethuraman M. G. (2016). Green synthesis of silver nanoparticles using Terminalia cuneata and its catalytic action in reduction of direct yellow-12 dye. Pectrochimica Acta Part A 161 122–129. 10.1016/j.saa.2016.02.044 [ PubMed ] [ CrossRef ] [ Google Scholar ]
- Elliott J. A., Shibuta Y., Amara H., Bichara C., Neyts E. C. (2013). Atomistic modelling of CVD synthesis of carbon nanotubes and graphene. Nanoscale 5 6662–6676. 10.1039/c3nr01925j [ PubMed ] [ CrossRef ] [ Google Scholar ]
- Erasmus M., Cason E. D., Van Marwijk J., Botes E., Gericke M., Van Heerden E. (2014). Gold nanoparticle synthesis using the thermophilic bacterium Thermus scotoductus SA-01 and the purification and characterization of its unusual gold reducing protein. Gold Bull. 47 245–253. [ Google Scholar ]
- Eroglu E., Chen X., Bradshaw M., Agarwal V., Zou J., Stewart S. G., et al. (2013). Biogenic production of palladium nanocrystals using microalgae and their immobilization on chitosan nanofibers for catalytic applications. RSC Adv. 3 1009–1012. [ Google Scholar ]
- Essajai R., Benhouria Y., Rachadi A., Qjani M., Mzerd A., Hassanain N. (2019). Shape-dependent structural and magnetic properties of Fe nanoparticles studied through simulation methods. RSC Adv. 9 22057–22063. 10.1039/c9ra03047f [ PMC free article ] [ PubMed ] [ CrossRef ] [ Google Scholar ]
- Falke S., Betzel C. (2019). “ Dynamic light scattering (DLS) ,” in Radiation in Bioanalysis , eds Pereira A. S., Tavares P., Limão-Vieira P. (Berlin: Springer; ), 173–193. [ Google Scholar ]
- Farrell D., Majetich S. A., Wilcoxon J. P. (2003). Preparation and characterization of monodisperse Fe nanoparticles. J. Phys. Chem. B 107 11022–11030. [ Google Scholar ]
- Feng L., Xuan Z., Ma J., Chen J., Cui D., Su C., et al. (2015). Preparation of gold nanorods with different aspect ratio and the optical response to solution refractive index. J. Exp. Nanosci. 10 258–267. [ Google Scholar ]
- Ghorbani H. R., Mehr F. P., Pazoki H., Rahmani B. M. (2015). Synthesis of ZnO nanoparticles by precipitation method. Orient. J. Chem. 31 1219–1221. [ Google Scholar ]
- Ghosh S., Ahmad R., Zeyaullah M., Khare S. K. (2021). Microbial nano-factories: synthesis and biomedical applications. Front. Chem. 9 : 194 . 10.3389/fchem.2021.626834 [ PMC free article ] [ PubMed ] [ CrossRef ] [ Google Scholar ]
- Giljohann D. A., Seferos D. S., Daniel W. L., Massich M. D., Patel P. C., Mirkin C. A. (2020). Gold nanoparticles for biology and medicine. Spherical Nucleic Acids 49 3280–3294. [ PMC free article ] [ PubMed ] [ Google Scholar ]
- Giurlani W., Innocenti M., Lavacchi A. (2018). X-ray microanalysis of precious metal thin films: thickness and composition determination. Coatings 8 : 84 . [ Google Scholar ]
- Gloria E. C., Ederley V., Gladis M., César H., Jaime O., Oscar A., et al. (2017). “ Synthesis of silver nanoparticles (AgNPs) with antibacterial activity ,” in Proceedings of the Journal of Physics: Conference Series , (United Kingdom: IOP Publishing; ), 012023. [ Google Scholar ]
- Gorrasi G., Sorrentino A. (2015). Mechanical milling as a technology to produce structural and functional bio-nanocomposites. Green Chem. 17 2610–2625. [ Google Scholar ]
- Govindarajan M., Rajeswary M., Veerakumar K., Muthukumaran U., Hoti S., Benelli G. (2016). Green synthesis and characterization of silver nanoparticles fabricated using Anisomeles indica: mosquitocidal potential against malaria, dengue and Japanese encephalitis vectors. Exp. Parasitol. 161 40–47. 10.1016/j.exppara.2015.12.011 [ PubMed ] [ CrossRef ] [ Google Scholar ]
- Graf C., Vossen D. L., Imhof A., Van Blaaderen A. (2003). A general method to coat colloidal particles with silica. Langmuir 19 6693–6700. [ PubMed ] [ Google Scholar ]
- Greczynski G., Hultman L. (2020). X-ray photoelectron spectroscopy: towards reliable binding energy referencing. Progr. Mater. Sci. 107 : 100591 . [ Google Scholar ]
- Guo D., Xie G., Luo J. (2013). Mechanical properties of nanoparticles: basics and applications. J. Phys. D 47 : 013001 . [ Google Scholar ]
- Guo W., Pleixats R., Shafir A. (2015). Water-soluble gold nanoparticles: from catalytic selective Nitroarene reduction in water to refractive index sensing. Chem. An Asian J. 10 2437–2443. 10.1002/asia.201500290 [ PubMed ] [ CrossRef ] [ Google Scholar ]
- Gwynne K. (2020). Enhancement of the Photostability of Blue Phosphorescence Using Plasmonic Surfaces. New Brunswick, NJ: Rutgers University-School of Graduate Studies. [ Google Scholar ]
- Haasch R. T. (2014). “ X-ray photoelectron spectroscopy (XPS) and auger electron spectroscopy (AES) ,” in Practical Materials Characterization , Ed. Sardela M. (Berlin: Springer; ), 93–132. [ Google Scholar ]
- Hasan S. (2015). A review on nanoparticles: their synthesis and types. Res. J. Recent Sci. 2277 : 2502 . [ Google Scholar ]
- Holder C. F., Schaak R. E. (2019). Tutorial on Powder X-ray Diffraction for Characterizing Nanoscale Materials. Washington, DC: ACS Publications. [ PubMed ] [ Google Scholar ]
- Hollamby M. J., Eastoe J., Chemelli A., Glatter O., Rogers S., Heenan R. K., et al. (2010). Separation and purification of nanoparticles in a single step. Langmuir 26 6989–6994. 10.1021/la904225k [ PubMed ] [ CrossRef ] [ Google Scholar ]
- Hoo C. M., Starostin N., West P., Mecartney M. L. (2008). A comparison of atomic force microscopy (AFM) and dynamic light scattering (DLS) methods to characterize nanoparticle size distributions. J. Nanopart. Res. 10 89–96. [ Google Scholar ]
- Hortin J., Anderson A., Britt D., Jacobson A., Mclean J. (2020). Copper oxide nanoparticle dissolution at alkaline pH is controlled by dissolved organic matter: influence of soil-derived organic matter, wheat, bacteria, and nanoparticle coating. Environ. Sci. 7 2618–2631. [ Google Scholar ]
- Hoseinzadeh E., Makhdoumi P., Taha P., Hossini H., Stelling J., Amjad Kamal M. (2017). A review on nano-antimicrobials: metal nanoparticles, methods and mechanisms. Curr. Drug Metab. 18 120–128. [ PubMed ] [ Google Scholar ]
- Hulkoti N. I., Taranath T. (2014). Biosynthesis of nanoparticles using microbes—a review. Colloids Surf. B Biointerf. 121 474–483. 10.1016/j.colsurfb.2014.05.027 [ PubMed ] [ CrossRef ] [ Google Scholar ]
- Islam F., Shohag S., Uddin M. J., Islam M. R., Nafady M. H., Akter A., et al. (2022). Exploring the journey of zinc oxide nanoparticles (ZnO-NPs) toward biomedical applications. Materials 15 : 2160 . 10.3390/ma15062160 [ PMC free article ] [ PubMed ] [ CrossRef ] [ Google Scholar ]
- Jadoun S., Arif R., Jangid N. K., Meena R. K. (2021). Green synthesis of nanoparticles using plant extracts: A review. Environ. Chem. Lett. 19 355–374. [ Google Scholar ]
- Jamkhande P. G., Ghule N. W., Bamer A. H., Kalaskar M. G. (2019). Metal nanoparticles synthesis: An overview on methods of preparation, advantages and disadvantages, and applications. J. Drug Deliv. Sci. Technol. 53 101174 . [ Google Scholar ]
- Jana N. R., Earhart C., Ying J. Y. (2007). Synthesis of water-soluble and functionalized nanoparticles by silica coating. Chem. Mater. 19 5074–5082. [ Google Scholar ]
- Jaskulski D., Jaskulska I., Majewska J., Radziemska M., Bilgin A., Brtnicky M. (2022). Silver Nanoparticles (AgNPs) in urea solution in laboratory tests and field experiments with crops and vegetables. Materials 15 : 870 . 10.3390/ma15030870 [ PMC free article ] [ PubMed ] [ CrossRef ] [ Google Scholar ]
- Jayaraman V., Ghosh S., Sengupta A., Srivastava S., Sonawat H., Narayan P. K. (2014). Identification of biochemical differences between different forms of male infertility by nuclear magnetic resonance (NMR) spectroscopy. J. Assist. Reproduct. Genet. 31 1195–1204. 10.1007/s10815-014-0282-4 [ PMC free article ] [ PubMed ] [ CrossRef ] [ Google Scholar ]
- Jena J., Pradhan N., Nayak R. R., Dash B. P., Sukla L. B., Panda P. K., et al. (2014). Microalga Scenedesmus sp.: a potential low-cost green machine for silver nanoparticle synthesis. J. Microbiol. Biotechnol. Adv. 24 522–533. 10.4014/jmb.1306.06014 [ PubMed ] [ CrossRef ] [ Google Scholar ]
- Jiang H.-L., Xu Q. (2011). Catalytic hydrolysis of ammonia borane for chemical hydrogen storage. Catal. Today 170 56–63. [ Google Scholar ]
- Joh D.-W., Jung T.-K., Lee H.-S., Kim D.-H. (2013). Synthesis of nanoparticles using electrical explosion of Ni wire in Pt solution. J. Nanosci. Nanotechnol. 13 6092–6094. 10.1166/jnn.2013.7677 [ PubMed ] [ CrossRef ] [ Google Scholar ]
- Kahle M., Kleber M., Jahn R. (2002). Review of XRD-based quantitative analyses of clay minerals in soils: the suitability of mineral intensity factors. Geoderma 109 191–205. [ Google Scholar ]
- Kalaiyarasu T., Karthi N., Sharmila G. V., Manju V. (2016). In vitro assessment of antioxidant and antibacterial activity of green synthesized silver nanoparticles from Digitaria radicosa leaves. Asian J. Pharm. Clin. Res. 9 297–302. [ Google Scholar ]
- Kayalvizhi T., Ravikumar S., Venkatachalam P. (2016). Green synthesis of metallic silver nanoparticles using Curculigo orchioides rhizome extracts and evaluation of its antibacterial, larvicidal, and anticancer activity. J. Environ. Eng. 142 : C4016002 . [ Google Scholar ]
- Khan A., Rashid R., Murtaza G., Zahra A. (2014). Gold nanoparticles: synthesis and applications in drug delivery. Trop. J. Pharm. Res. 13 1169–1177. [ Google Scholar ]
- Khan I., Saeed K., Khan I. (2019). Nanoparticles: Properties, applications and toxicities. Arab. J. Chem. 12 908–931. [ Google Scholar ]
- Khanna P., Kaur A., Goyal D. (2019). Algae-based metallic nanoparticles: Synthesis, characterization and applications. J. Microbiol Methods 163 : 105656 . 10.1016/j.mimet.2019.105656 [ PubMed ] [ CrossRef ] [ Google Scholar ]
- Kharat S. N., Mendhulkar V. D. (2016). Synthesis, characterization and studies on antioxidant activity of silver nanoparticles using Elephantopus scaber leaf extract. Mater. Sci. Eng. C 62 719–724. 10.1016/j.msec.2016.02.024 [ PubMed ] [ CrossRef ] [ Google Scholar ]
- Kim J.-H., Sheikh F. A., Ju H. W., Park H. J., Moon B. M., Lee O. J., et al. (2014). 3D silk fibroin scaffold incorporating titanium dioxide (TiO2) nanoparticle (NPs) for tissue engineering. Int. J. Biol. Macromol. 68 158–168. 10.1016/j.ijbiomac.2014.04.045 [ PubMed ] [ CrossRef ] [ Google Scholar ]
- Kohl H., Reimer L. (2008). Transmission Electron Microscopy. Berlin: Springer Series in Optical Sciences, 36. [ Google Scholar ]
- Kokarneswaran M., Selvaraj P., Ashokan T., Perumal S., Sellappan P., Murugan K. D., et al. (2020). Discovery of carbon nanotubes in sixth century BC potteries from Keeladi, India. Sci. Rep. 10 1–6. 10.1038/s41598-020-76720-z [ PMC free article ] [ PubMed ] [ CrossRef ] [ Google Scholar ]
- Kopittke P. M., Lombi E., Wang P., Schjoerring J. K., Husted S. (2019). Nanomaterials as fertilizers for improving plant mineral nutrition and environmental outcomes. Environ. Sci. 6 3513–3524. 10.3390/biology10111123 [ PMC free article ] [ PubMed ] [ CrossRef ] [ Google Scholar ]
- Kora A. J., Rastogi L. (2018). Peroxidase activity of biogenic platinum nanoparticles: A colorimetric probe towards selective detection of mercuric ions in water samples. Sens. Actuators B Chem. 254 690–700. [ Google Scholar ]
- Kreizer M., Ratner D., Liberzon A. (2010). Real-time image processing for particle tracking velocimetry. Exp. Fluids 48 105–110. [ Google Scholar ]
- Krithiga N., Jayachitra A., Rajalakshmi A. (2013). Synthesis, characterization and analysis of the effect of copper oxide nanoparticles in biological systems. Ind. J. Ns 1 6–15. [ Google Scholar ]
- Kumar R., Singh R. K., Dubey P. K., Kumar P., Tiwari R. S., Oh I.-K. (2013). Pressure-dependent synthesis of high-quality few-layer graphene by plasma-enhanced arc discharge and their thermal stability. J. Nanopart. Res. 15 1–10. [ Google Scholar ]
- Kumar S. S., Venkateswarlu P., Rao V. R., Rao G. N. (2013). Synthesis, characterization and optical properties of zinc oxide nanoparticles. Int. Nano Lett. 3 1–6. [ Google Scholar ]
- Kumar V., Yadav S. K. (2009). Plant-mediated synthesis of silver and gold nanoparticles and their applications. J. Chem. Technol. Biotechnol. 84 151–157. [ Google Scholar ]
- Kumar V., Bano D., Mohan S., Singh D. K., Hasan S. H. (2016). Sunlight-induced green synthesis of silver nanoparticles using aqueous leaf extract of Polyalthia longifolia and its antioxidant activity. Mater. Lett. 181 371–377. [ Google Scholar ]
- Kumari S. C., Dhand V., Padma P. N. (2021). Green synthesis of metallic nanoparticles: a review. Nanomaterials 2021 259–281. [ Google Scholar ]
- Lam E., Luong J. H. (2014). Carbon materials as catalyst supports and catalysts in the transformation of biomass to fuels and chemicals. ACS Catal. 4 3393–3410. [ Google Scholar ]
- Lara P., Philippot K. (2014). The hydrogenation of nitroarenes mediated by platinum nanoparticles: an overview. Catal. Sci. Technol. 4 2445–2465. [ Google Scholar ]
- Lerner M. I., Glazkova E. A., Lozhkomoev A. S., Svarovskaya N. V., Bakina O. V., Pervikov A. V., et al. (2016). Synthesis of Al nanoparticles and Al/AlN composite nanoparticles by electrical explosion of aluminum wires in argon and nitrogen. Powder Technol. 295 307–314. [ Google Scholar ]
- Lewczuk B., Szyryńska N. (2021). Field-emission scanning electron microscope as a tool for large-area and large-volume ultrastructural studies. Animals 11 : 3390 . 10.3390/ani11123390 [ PMC free article ] [ PubMed ] [ CrossRef ] [ Google Scholar ]
- Li G., He D., Qian Y., Guan B., Gao S., Cui Y., et al. (2011). Fungus-mediated green synthesis of silver nanoparticles using Aspergillus terreus. Int. J. Mol. Sci. 13 466–476. 10.3390/ijms13010466 [ PMC free article ] [ PubMed ] [ CrossRef ] [ Google Scholar ]
- Li N., Zhao P., Astruc D. (2014). Anisotropic gold nanoparticles: synthesis, properties, applications, and toxicity. Angewand. Chem. Int. Edn. 53 1756–1789. [ PubMed ] [ Google Scholar ]
- Li T., Senesi A. J., Lee B. (2016). Small angle X-ray scattering for nanoparticle research. Chem. Rev. 116 11128–11180. 10.1021/acs.chemrev.5b00690 [ PubMed ] [ CrossRef ] [ Google Scholar ]
- Li X., Xu H., Chen Z.-S., Chen G. (2011). Biosynthesis of nanoparticles by microorganisms and their applications. J. Nanomater. 2011 : 270974 . [ Google Scholar ]
- Luangpipat T., Beattie I. R., Chisti Y., Haverkamp R. G. (2011). Gold nanoparticles produced in a microalga. J. Nanopart. Res. 13 6439–6445. [ Google Scholar ]
- Lyon L. A., Keating C. D., Fox A. P., Baker B. E., He L., Nicewarner S. R., et al. (1998). Raman spectroscopy. Anal. Chem. 70 341–362. [ PubMed ] [ Google Scholar ]
- Lyu H., Gao B., He F., Ding C., Tang J., Crittenden J. C. (2017). Ball-milled carbon nanomaterials for energy and environmental applications. ACS Sust. Chem. Eng. 5 9568–9585. 10.1016/j.biortech.2020.123613 [ PubMed ] [ CrossRef ] [ Google Scholar ]
- Machac P., Cichon S., Lapcak L., Fekete L. (2020). Graphene prepared by chemical vapour deposition process. Graph. Technol. 5 9–17. [ Google Scholar ]
- Madathil A. N. P., Vanaja K., Jayaraj M. (2007). “ Synthesis of ZnO nanoparticles by hydrothermal method ,” in Nanophotonic materials IV , eds Gaburro Z., Cabrini S. (Bellingham, WA: SPIE; ), 47–55. [ Google Scholar ]
- Maharani V., Sundaramanickam A., Balasubramanian T. J. E. (2016). In vitro anticancer activity of silver nanoparticle synthesized by Escherichia coli VM1 isolated from marine sediments of Ennore southeast coast of India. Enzyme Microb. Technol. 95 146–154. 10.1016/j.enzmictec.2016.09.008 [ PubMed ] [ CrossRef ] [ Google Scholar ]
- Majeed Khan M. A., Kumar S., Ahamed M., Alrokayan S. A., Alsalhi M. S. (2011). Structural and thermal studies of silver nanoparticles and electrical transport study of their thin films. Nanosc. Res. Lett. 6 1–8. [ PMC free article ] [ PubMed ] [ Google Scholar ]
- Malhotra S. P. K., Alghuthaymi M. A. (2022). Biomolecule-assisted biogenic synthesis of metallic nanoparticles. Agri-Waste Microb. Product. Sust. Nanomater. 2022 139–163. [ Google Scholar ]
- Mathew L., Chandrasekaran N., Mukherjee A. (2010). “ Biomimetic synthesis of nanoparticles: science, technology & applicability ,” Biomimetics learning from nature , Ed. Mukherjee A. (Norderstedt: Books on Demand; ). [ Google Scholar ]
- Mishra A., Tripathy S. K., Wahab R., Jeong S.-H., Hwang I., Yang Y.-B., et al. (2011). Microbial synthesis of gold nanoparticles using the fungus Penicillium brevicompactum and their cytotoxic effects against mouse mayo blast cancer C 2 C 12 cells. Appl. Microbiol. Biotechnol. Adv. 92 617–630. 10.1007/s00253-011-3556-0 [ PubMed ] [ CrossRef ] [ Google Scholar ]
- Mittal A., Chisti Y. (2013). Synthesis of metallic nanoparticles using plant extracts. Biotechnol. Adv. 31 346–356. [ PubMed ] [ Google Scholar ]
- Moghaddam A. B., Nazari T., Badraghi J., Kazemzad M. (2009). Synthesis of ZnO nanoparticles and electrodeposition of polypyrrole/ZnO nanocomposite film. Int. J. Electrochem. Sci. 4 247–257. [ Google Scholar ]
- Mohanpuria P., Rana N. K., Yadav S. K. (2008). Biosynthesis of nanoparticles: technological concepts and future applications. J. Nanopart. Res. 10 507–517. [ Google Scholar ]
- Mohd Yusof H., Mohamad R., Zaidan U. H., Rahman A. (2019). Microbial synthesis of zinc oxide nanoparticles and their potential application as an antimicrobial agent and a feed supplement in animal industry: a review. J. Anim. Sci. Biotechnol. 10 1–22. 10.1186/s40104-019-0368-z [ PMC free article ] [ PubMed ] [ CrossRef ] [ Google Scholar ]
- Morsi M., Abdelrazek E., Ramadan R., Elashmawi I., Rajeh A. (2022). Structural, optical, mechanical, and dielectric properties studies of carboxymethyl cellulose/polyacrylamide/lithium titanate nanocomposites films as an application in energy storage devices. Polymer Test. 114 107705 . [ Google Scholar ]
- Mott D., Galkowski J., Wang L., Luo J., Zhong C.-J. (2007). Synthesis of size-controlled and shaped copper nanoparticles. Langmuir 23 5740–5745. [ PubMed ] [ Google Scholar ]
- Mukherjee P., Ahmad A., Mandal D., Senapati S., Sainkar S. R., Khan M. I., et al. (2001). Fungus-mediated synthesis of silver nanoparticles and their immobilization in the mycelial matrix: a novel biological approach to nanoparticle synthesis. Nano Lett. 1 515–519. [ Google Scholar ]
- Muñoz-García J., Vázquez L., Cuerno R., Sánchez-García J. A., Castro M., Gago R. (2009). “ Self-organized surface nanopatterning by ion beam sputtering ,” in Toward Functional Nanomaterials , Ed. Wang Z. M. (Berlin: Springer; ), 323–398. [ Google Scholar ]
- Naraginti S., Kumari P. L., Das R. K., Sivakumar A., Patil S. H., Andhalkar V. V. (2016). Amelioration of excision wounds by topical application of green synthesized, formulated silver and gold nanoparticles in albino Wistar rats. Mater. Sci. Eng. C 62 293–300. 10.1016/j.msec.2016.01.069 [ PubMed ] [ CrossRef ] [ Google Scholar ]
- Narayanan K. B., Sakthivel N. (2010). Biological synthesis of metal nanoparticles by microbes. Adv. Colloid Interf. Sci. 156 1–13. [ PubMed ] [ Google Scholar ]
- Nayak D., Ashe S., Rauta P. R., Kumari M., Nayak B. (2016). Bark extract mediated green synthesis of silver nanoparticles: evaluation of antimicrobial activity and antiproliferative response against osteosarcoma. Mater. Sci. Eng. C 58 44–52. 10.1016/j.msec.2015.08.022 [ PubMed ] [ CrossRef ] [ Google Scholar ]
- Ndolomingo M. J., Meijboom R. (2016). Determination of the surface area and sizes of supported copper nanoparticles through organothiol adsorption—Chemisorption. Appl. Surf. Sci. 390 224–235. [ Google Scholar ]
- Newbury D. E., Ritchie N. W. (2013). Is scanning electron microscopy/energy dispersive X-ray spectrometry (SEM/EDS) quantitative? Scanning 35 141–168. 10.1002/sca.21041 [ PubMed ] [ CrossRef ] [ Google Scholar ]
- Nguyen K. T., Menon J. U., Jadeja P. V., Tambe P. P., Vu K., Yuan B. (2013). Nanomaterials for photo-based diagnostic and therapeutic applications. Theranostics 3 152–166. [ PMC free article ] [ PubMed ] [ Google Scholar ]
- Nowack B., Krug H. F., Height M. (2011). 120 Years of Nanosilver History: Implications for Policy Makers. Washington, DC: ACS Publications. [ PubMed ] [ Google Scholar ]
- Önal E. S., Yatkın T., Aslanov T., Ergüt M., Özer A. (2019). Biosynthesis and characterization of iron nanoparticles for effective adsorption of Cr (VI). Int. J. Chem. Eng. 2019 : 2716423 . [ Google Scholar ]
- Ostermann R., Cravillon J., Weidmann C., Wiebcke M., Smarsly B. M. (2011). Metal–organic framework nanofibers via electrospinning. Chem. Commun. 47 442–444. [ PubMed ] [ Google Scholar ]
- Parashar M., Shukla V. K., Singh R. (2020). Metal oxides nanoparticles via sol–gel method: a review on synthesis, characterization and applications. J. Mater. Sci. 31 3729–3749. [ Google Scholar ]
- Park C. Y., Choi B. (2019). Enhanced light extraction from bottom emission oleds by high refractive index nanoparticle scattering layer. Nanomaterials 9 : 1241 . 10.3390/nano9091241 [ PMC free article ] [ PubMed ] [ CrossRef ] [ Google Scholar ]
- Patil M. P., Kim G.-D. (2018). Marine microorganisms for synthesis of metallic nanoparticles and their biomedical applications. Colloids Surf. B Biointerf. 172 487–495. [ PubMed ] [ Google Scholar ]
- Patil M. P., Ngabire D., Thi H. H. P., Kim M.-D., Kim G.-D. (2017). Eco-friendly synthesis of gold nanoparticles and evaluation of their cytotoxic activity on cancer cells. J. Clust. Sci. 28 119–132. [ Google Scholar ]
- Patil N., Bhaskar R., Vyavhare V., Dhadge R., Khaire V., Patil Y. (2021). Overview on methods of synthesis of nanoparticles. Int. J. Curr. Pharm. Res. 13 11–16. [ Google Scholar ]
- Patois E., Capelle M., Palais C., Gurny R., Arvinte T. (2012). Evaluation of nanoparticle tracking analysis (NTA) in the characterization of therapeutic antibodies and seasonal influenza vaccines: pros and cons. J. Drug Deliv. Sci. Technol. 22 427–433. [ Google Scholar ]
- Paul B., Bhuyan B., Purkayastha D. D., Dhar S. S. (2016). Photocatalytic and antibacterial activities of gold and silver nanoparticles synthesized using biomass of Parkia roxburghii leaf. J. Photochem. Photobiol. B Biol. 154 1–7. 10.1016/j.jphotobiol.2015.11.004 [ PubMed ] [ CrossRef ] [ Google Scholar ]
- Pérez-Lorenzo M. (2012). Palladium nanoparticles as efficient catalysts for Suzuki cross-coupling reactions. J. Phys. Chem. Lett. 3 167–174. [ Google Scholar ]
- Pérez-Tijerina E., Pinilla M. G., Mejía-Rosales S., Ortiz-Méndez U., Torres A., José-Yacamán M. (2008). Highly size-controlled synthesis of Au/Pd nanoparticles by inert-gas condensation. Faraday Discuss. 138 353–362. 10.1039/b705913m [ PubMed ] [ CrossRef ] [ Google Scholar ]
- Phull A.-R., Abbas Q., Ali A., Raza H., Zia M., Haq I.-U. (2016). Antioxidant, cytotoxic and antimicrobial activities of green synthesized silver nanoparticles from crude extract of Bergenia ciliata. Fut. J. Pharm. Sci. 2 31–36. [ Google Scholar ]
- Pimpin A., Srituravanich W. (2012). Review on micro-and nanolithography techniques and their applications. Eng. J. 16 37–56. [ Google Scholar ]
- Pradeep T. (2009). Noble metal nanoparticles for water purification: a critical review. Thin Solid Films 517 6441–6478. [ Google Scholar ]
- Praseptiangga D., Zahara H. L., Widjanarko P. I., Joni I. M., Panatarani C. (2020). Preparation and FTIR spectroscopic studies of SiO2-ZnO nanoparticles suspension for the development of carrageenan-based bio-nanocomposite film. 100005 . [ Google Scholar ]
- Pugazhendhi S., Sathya P., Palanisamy P., Gopalakrishnan R. (2016). Synthesis of silver nanoparticles through green approach using Dioscorea alata and their characterization on antibacterial activities and optical limiting behavior. J. Photochem. Photobiol. B Biol. 159 155–160. 10.1016/j.jphotobiol.2016.03.043 [ PubMed ] [ CrossRef ] [ Google Scholar ]
- Qi P., Zhang D., Wan Y. (2013). Sulfate-reducing bacteria detection based on the photocatalytic property of microbial synthesized ZnS nanoparticles. Anal. Chim. Acta 800 65–70. 10.1016/j.aca.2013.09.015 [ PubMed ] [ CrossRef ] [ Google Scholar ]
- Rad A. G., Abbasi H., Afzali M. H. (2011). Gold nanoparticles: synthesising, characterizing and reviewing novel application in recent years. Phys. Proc. 22 203–208. [ Google Scholar ]
- Rahmati-Abkenar M., Manteghian M. (2020). Effect of silver nanoparticles on the solubility of methane and ethane in water. J. Nat. Gas Sci. Eng. 82 : 103505 . [ Google Scholar ]
- Rajeshkumar S., Ponnanikajamideen M., Malarkodi C., Malini M., Annadurai G. (2014). Microbe-mediated synthesis of antimicrobial semiconductor nanoparticles by marine bacteria. J. Nanostruct. Chem. 4 1–7. [ Google Scholar ]
- Rajkuberan C., Prabukumar S., Sathishkumar G., Wilson A., Ravindran K., Sivaramakrishnan S. (2017). Facile synthesis of silver nanoparticles using Euphorbia antiquorum L. latex extract and evaluation of their biomedical perspectives as anticancer agents. J. Saudi Chem. Soc. 21 911–919. [ Google Scholar ]
- Ramesh P., Kokila T., Geetha D. (2015). Plant mediated green synthesis and antibacterial activity of silver nanoparticles using Emblica officinalis fruit extract. Spectrochim. Acta Part A 142 339–343. 10.1016/j.saa.2015.01.062 [ PubMed ] [ CrossRef ] [ Google Scholar ]
- Rangel-Olivares F. R., Arce-Estrada E. M., Cabrera-Sierra R. (2021). Synthesis and characterization of polyaniline-based polymer nanocomposites as anti-corrosion coatings. Coatings 11 : 653 . [ Google Scholar ]
- Rao N. H., Lakshmidevi N., Pammi S., Kollu P., Ganapaty S., Lakshmi P. (2016). Green synthesis of silver nanoparticles using methanolic root extracts of Diospyros paniculata and their antimicrobial activities. Mater. Sci. Eng. C 62 553–557. 10.1016/j.msec.2016.01.072 [ PubMed ] [ CrossRef ] [ Google Scholar ]
- Rassaei L., Marken F., Sillanpää M., Amiri M., Cirtiu C. M., Sillanpää M. (2011). Nanoparticles in electrochemical sensors for environmental monitoring. TrAC Trends Anal. Chem. 30 1704–1715. [ Google Scholar ]
- Rocha F. S., Gomes A. J., Lunardi C. N., Kaliaguine S., Patience G. S. (2018). Experimental methods in chemical engineering: Ultraviolet visible spectroscopy—UV-Vis. Can. J. Chem. Eng. 96 2512–2517. [ Google Scholar ]
- Saini P., Saha S. K., Roy P., Chowdhury P., Babu S. P. S. (2016). Evidence of reactive oxygen species (ROS) mediated apoptosis in Setaria cervi induced by green silver nanoparticles from Acacia auriculiformis at a very low dose. Exp. Parasitol. 160 39–48. 10.1016/j.exppara.2015.11.004 [ PubMed ] [ CrossRef ] [ Google Scholar ]
- Saldarriaga J. F., Aguado R., Pablos A., Amutio M., Olazar M., Bilbao J. (2015). Fast characterization of biomass fuels by thermogravimetric analysis (TGA). Fuel 140 744–751. [ Google Scholar ]
- Salem S. S., Fouda A. (2021). Green synthesis of metallic nanoparticles and their prospective biotechnological applications: an overview. Biol. Trace Element Res. 199 344–370. 10.1007/s12011-020-02138-3 [ PubMed ] [ CrossRef ] [ Google Scholar ]
- Salopek B., Krasic D., Filipovic S. (1992). Measurement and application of zeta-potential. Rudarsko-Geolosko-Naftni Zbornik 4 : 147 . [ Google Scholar ]
- Saw M. J., Ghosh B., Nguyen M. T., Jirasattayaporn K., Kheawhom S., Shirahata N., et al. (2019). High aspect ratio and post-processing free silver nanowires as top electrodes for inverted-structured photodiodes. ACS Omega 4 13303–13308. 10.1021/acsomega.9b01479 [ PMC free article ] [ PubMed ] [ CrossRef ] [ Google Scholar ]
- Schröfel A., Kratošová G., Šafa r ̄ ík I., Šafa r ̄ íková M., Raška I., Shor L. M. (2014). Applications of biosynthesized metallic nanoparticles–a review. Acta Biomater. 10 4023–4042. [ PubMed ] [ Google Scholar ]
- Shenashen M. A., El-Safty S. A., Elshehy E. A. (2014). Synthesis, morphological control, and properties of silver nanoparticles in potential applications. Part. Part. Syst. Char. 31 293–316. [ Google Scholar ]
- Shi Z., Lian Y., Liao F. H., Zhou X., Gu Z., Zhang Y., et al. (2000). Large scale synthesis of single-wall carbon nanotubes by arc-discharge method. J. Phys. Chem. Solids 61 1031–1036. 10.1166/jnn.2001.012 [ PubMed ] [ CrossRef ] [ Google Scholar ]
- Siddiqi K. S., Husen A. (2016). Green synthesis, characterization and uses of palladium/platinum nanoparticles. Nanosc. Res. Lett. 11 1–13. 10.1186/s11671-016-1695-z [ PMC free article ] [ PubMed ] [ CrossRef ] [ Google Scholar ]
- Siddique S., Chow J. C. (2020). Gold nanoparticles for drug delivery and cancer therapy. Appl. Sci. 10 : 3824 . [ Google Scholar ]
- Sigmund W., Yuh J., Park H., Maneeratana V., Pyrgiotakis G., Daga A. (2006). Processing and structure relationships in electrospinning of ceramic fiber systems. J. Am. Ceramic Soc. 89 395–407. [ Google Scholar ]
- Singh R. P., Shukla V. K., Yadav R. S., Sharma P. K., Singh P. K., Pandey A. C. (2011). Biological approach of zinc oxide nanoparticles formation and its characterization. Adv. Mater. Lett. 2 313–317. [ Google Scholar ]
- Siwach O. P., Sen P. (2008). Synthesis and study of fluorescence properties of Cu nanoparticles. J. Nanopart. Res. 10 107–114. [ Google Scholar ]
- Slavin Y. N., Asnis J., Häfeli U. O., Bach H. (2017). Metal nanoparticles: understanding the mechanisms behind antibacterial activity. J. Nanobiotechnol. 15 1–20. [ PMC free article ] [ PubMed ] [ Google Scholar ]
- Song U., Kim J. (2020). Zinc oxide nanoparticles: a potential micronutrient fertilizer for horticultural crops with little toxicity. Horticult. Environ. Biotechnol. 61 625–631. [ Google Scholar ]
- Soni N., Sonam P. (2014). Green nanoparticles for mosquito control. Sci. World J. 2014 1–6. [ PMC free article ] [ PubMed ] [ Google Scholar ]
- Sowani H., Mohite P., Munot H., Shouche Y., Bapat T., Kumar A. R., et al. (2016). Green synthesis of gold and silver nanoparticles by an actinomycete Gordonia amicalis HS-11: mechanistic aspects and biological application. Process Biochem. 51 374–383. [ Google Scholar ]
- Sriram M. I., Kalishwaralal K., Barathmanikanth S., Gurunathani S. (2012). Size-based cytotoxicity of silver nanoparticles in bovine retinal endothelial cells. Nanosci. Methods 1 56–77. [ Google Scholar ]
- Stepanov A. L., Nuzhdin V. I., Valeev V. F., Kreibig U. (2011). “ Optical properties of metal nanoparticles ,” in Proceedings of the ICONO 2010: International Conference on Coherent and Nonlinear Optics , (Bellingham, WA: SPIE; ), 543–552. [ Google Scholar ]
- Su S. S., Chang I. (2018). “ Review of production routes of nanomaterials ,” in Commercialization of nanotechnologies–a case study approach , eds Brabazon D., Pellicer E., Zivic F., Sort J., Baró M. D., Grujovic N., Choy K.-L. (Berlin: Springer; ), 15–29. [ Google Scholar ]
- Sugihartono I., Dianisya D., Isnaeni I. (2018). “ Crystal structure analyses of ZnO nanoparticles growth by simple wet chemical method ,” in Proceedings of the IOP Conference Series: Materials Science and Engineering , (Bristol: IOP Publishing; ), 012077. [ Google Scholar ]
- Sun T., Zhang Y. S., Pang B., Hyun D. C., Yang M., Xia Y. J. A. C. I. E. (2014). Engineered nanoparticles for drug delivery in cancer therapy. Angew. Chem. Int. Ed. Engl. 53 12320–12364. [ PubMed ] [ Google Scholar ]
- Tavakoli A. H., Maram P. S., Widgeon S. J., Rufner J., Van Benthem K., Ushakov S., et al. (2013). Amorphous alumina nanoparticles: structure, surface energy, and thermodynamic phase stability. J. Phys. Chem. C 117 17123–17130. 10.1021/jp405820g [ CrossRef ] [ Google Scholar ]
- Theron J., Eugene Cloete T., De Kwaadsteniet M. (2010). Current molecular and emerging nanobiotechnology approaches for the detection of microbial pathogens. Crit. Rev. Microbiol. 36 318–339. 10.3109/1040841X.2010.489892 [ PubMed ] [ CrossRef ] [ Google Scholar ]
- Thomas R., Janardhanan A., Varghese R. T., Soniya E., Mathew J., Radhakrishnan E. (2014). Antibacterial properties of silver nanoparticles synthesized by marine Ochrobactrum sp. Braz. J. Microbiol. 45 1221–1227. 10.1590/s1517-83822014000400012 [ PMC free article ] [ PubMed ] [ CrossRef ] [ Google Scholar ]
- Titus D., Samuel E. J. J., Roopan S. M. (2019). “ Nanoparticle characterization techniques ,” in Green synthesis, characterization and applications of nanoparticles , eds Shukla A., Iravani S. (Amsterdam: Elsevier; ), 303–319. 10.1016/B978-0-08-102579-6.00012-5 [ CrossRef ] [ Google Scholar ]
- Tran V., Wen X. (2014). “ Rapid prototyping technologies for tissue regeneration ,” in Rapid prototyping of biomaterials , Ed. Narayan R. (Sawston: Woodhead Publishing; ), 97–155. 10.1533/9780857097217.97 [ CrossRef ] [ Google Scholar ]
- Triana M. A., Hsiang E.-L., Zhang C., Dong Y., Wu S.-T. (2022). Luminescent nanomaterials for energy-efficient display and healthcare. ACS Energy Lett. 7 1001–1020. [ Google Scholar ]
- Uzair B., Liaqat A., Iqbal H., Menaa B., Razzaq A., Thiripuranathar G., et al. (2020). Green and cost-effective synthesis of metallic nanoparticles by algae: Safe methods for translational medicine. Bioengineering 7 : 129 . 10.3390/bioengineering7040129 [ PMC free article ] [ PubMed ] [ CrossRef ] [ Google Scholar ]
- Van Thai P., Abe S., Kosugi K., Saito N., Takahashi K., Sasaki T., et al. (2019). Size/shape control of gold nanoparticles synthesized by alternating current glow discharge over liquid: The role of pH. Mater. Res. Expr. 6 : 095074 . [ Google Scholar ]
- Velusamy P., Das J., Pachaiappan R., Vaseeharan B., Pandian K. (2015). Greener approach for synthesis of antibacterial silver nanoparticles using aqueous solution of neem gum ( Azadirachta indica L.). Indus. Crops Products 66 103–109. [ Google Scholar ]
- Wang P., Menzies N. W., Lombi E., Sekine R., Blamey F. P. C., Hernandez-Soriano M. C., et al. (2015). Silver sulfide nanoparticles (Ag2S-NPs) are taken up by plants and are phytotoxic. Nanotoxicology 9 1041–1049. 10.3109/17435390.2014.999139 [ PubMed ] [ CrossRef ] [ Google Scholar ]
- Wang Z., Li H., Tang F., Ma J., Zhou X. (2018). A facile approach for the preparation of nano-size zinc oxide in water/glycerol with extremely concentrated zinc sources. Nanosc. Res. Lett. 13 1–9. 10.1186/s11671-018-2616-0 [ PMC free article ] [ PubMed ] [ CrossRef ] [ Google Scholar ]
- Wiesendanger R., Güntherodt H.-J. (2013). Scanning tunneling microscopy III: theory of STM and related scanning probe methods. Berlin: Springer Science & Business Media. [ Google Scholar ]
- Xia Y., Xiao Z., Dou X., Huang H., Lu X., Yan R., et al. (2013). Green and facile fabrication of hollow porous MnO/C microspheres from microalgaes for lithium-ion batteries. ACS Nano 7 7083–7092. 10.1021/nn4023894 [ PubMed ] [ CrossRef ] [ Google Scholar ]
- Yadav R., Dwivedi S., Kumar S., Chaudhury A. (2010). Trends and perspectives of biosensors for food and environmental virology. Food Environ. Virol. 2 53–63. [ Google Scholar ]
- Yadav T. P., Yadav R. M., Singh D. P. (2012). Mechanical milling: a top down approach for the synthesis of nanomaterials and nanocomposites. Nanosci. Nanotechnol. 2 22–48. [ Google Scholar ]
- Yang W., Wang L., Mettenbrink E. M., Deangelis P. L., Wilhelm S. (2021). Nanoparticle toxicology. Annu. Rev. Pharmacol. Toxicol. 61 269–289. [ PubMed ] [ Google Scholar ]
- Ye Q., Chen W., Huang H., Tang Y., Wang W., Meng F., et al. (2020). Iron and zinc ions, potent weapons against multidrug-resistant bacteria. Appl. Microbiol. Biotechnol. 104 5213–5227. 10.1007/s00253-020-10600-4 [ PubMed ] [ CrossRef ] [ Google Scholar ]
- Yuan P., Ding X., Yang Y. Y., Xu Q. H. (2018). Metal nanoparticles for diagnosis and therapy of bacterial infection. Adv. Healthc. Mater. 7 : 1701392 . [ PubMed ] [ Google Scholar ]
- Zahra Z., Habib Z., Chung S., Badshah M. A. (2020). Exposure route of TiO2 NPs from industrial applications to wastewater treatment and their impacts on the agro-environment. Nanomaterials 10 : 1469 . 10.3390/nano10081469 [ PMC free article ] [ PubMed ] [ CrossRef ] [ Google Scholar ]
- Zan G., Wu Q. (2016). Biomimetic and bioinspired synthesis of nanomaterials/nanostructures. Adv. Mater. 28 2099–2147. [ PubMed ] [ Google Scholar ]
- Zhang X., Yan S., Tyagi R., Surampalli R. (2011). Synthesis of nanoparticles by microorganisms and their application in enhancing microbiological reaction rates. Chemosphere 82 489–494. [ PubMed ] [ Google Scholar ]
- Zheng Z., Zhang X., Carbo D., Clark C., Nathan C.-A., Lvov Y. (2010). Sonication-assisted synthesis of polyelectrolyte-coated curcumin nanoparticles. Langmuir 26 7679–7681. 10.1021/la101246a [ PMC free article ] [ PubMed ] [ CrossRef ] [ Google Scholar ]
- Zhou C., Wang Y., Du L., Yao H., Wang J., Luo G. (2016). Precipitation preparation of high surface area and porous nanosized ZnO by continuous gas-based impinging streams in unconfined space. Indus. Eng. Chem. Res. 55 11943–11949. [ Google Scholar ]
- Zhou M., Wei Z., Qiao H., Zhu L., Yang H., Xia T. (2009). Particle size and pore structure characterization of silver nanoparticles prepared by confined arc plasma. J. Nanomater. 2009 : 968058 . [ Google Scholar ]
- Zhuang J., Gentry R. W. (2011). “ Environmental application and risks of nanotechnology: a balanced view ,” in Biotechnology and Nanotechnology Risk Assessment: Minding and Managing the Potential Threats around Us , eds Ripp S., Henry T. (Washington, DC: ACS Publications; ), 41–67. 10.3390/ijerph16234848 [ CrossRef ] [ Google Scholar ]
- Zielińska A., Carreiró F., Oliveira A. M., Neves A., Pires B., Venkatesh D. N., et al. (2020). Polymeric nanoparticles: production, characterization, toxicology and ecotoxicology. Molecules 25 : 3731 . 10.3390/molecules25163731 [ PMC free article ] [ PubMed ] [ CrossRef ] [ Google Scholar ]
Thank you for visiting nature.com. You are using a browser version with limited support for CSS. To obtain the best experience, we recommend you use a more up to date browser (or turn off compatibility mode in Internet Explorer). In the meantime, to ensure continued support, we are displaying the site without styles and JavaScript.
- View all journals
Nanoparticles articles from across Nature Portfolio
Nanoparticles are particles that exist on a nanometre scale (i.e., below 100 nm in at least one dimension). They can possess physical properties such as uniformity, conductance or special optical properties that make them desirable in materials science and biology.
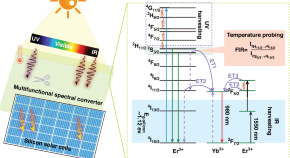
Multifunctional photon conversion materials for enhancing silicon solar cells
- Yiyan Zhang
- Guanying Chen
Latest Research and Reviews
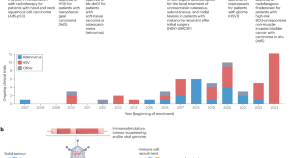
Nucleic acid-based drugs for patients with solid tumours
Nucleic acid-based therapies offer an alternative to traditional cancer treatment modalities, with promising data beginning to emerge. In this Review, the authors describe the design and development of nucleic acid-based therapies administered virally and non-virally, including discussions of the advantages and disadvantage of each approach, as well as the role of patient-specific factors such as the tumour microenvironment, and consider the most promising future research directions.
- Sebastian G. Huayamares
- David Loughrey
- Eric J. Sorscher
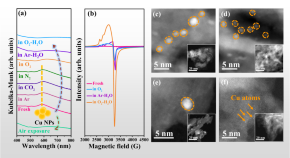
Water-assisted oxidative redispersion of Cu particles through formation of Cu hydroxide at room temperature
Redispersion of sintered metal species requires high temperatures in reactive atmospheres. Here, the authors report room temperature redispersion of supported Cu particles via formation of mobile hydroxylated Cu species induced by gaseous H 2 O and anchoring of the Cu species by surface OH groups.
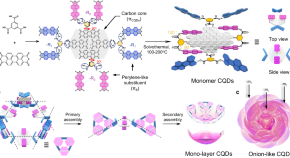
Onion-like multicolor thermally activated delayed fluorescent carbon quantum dots for efficient electroluminescent light-emitting diodes
Shi et al. report the synthesis of multicolour thermally activated delayed fluorescent carbon dots with 3D onion-like configuration to stabilise the triplet state and reduce the singlet-triplet energy gap. LEDs with EQE of 6.0–9.9% are achieved, a step further for efficient and stable displays.
- Louzhen Fan
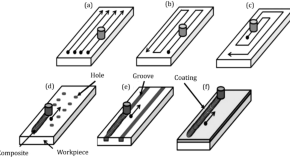
Evaluating the influence of various friction stir processing strategies on surface integrity of hybrid nanocomposite Al6061
- Navid Molla Ramezani
- Behnam Davoodi
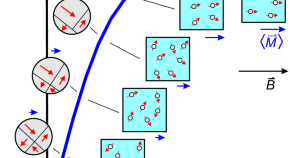
A comprehensive approach to characterize navigation instruments for magnetic guidance in biological systems
- Peter Blümler
- Fabian Raudzus
- Friederike Schmid
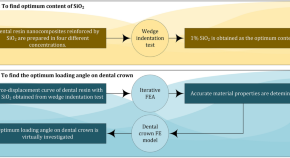
Experimental characterization and finite element investigation of SiO 2 nanoparticles reinforced dental resin composite
- Babak Jaleh
- Mohammad Kashfi
News and Comment
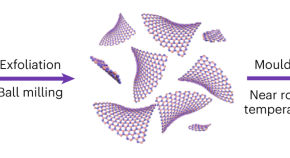
Nanosheets bulk up
- Alison Stoddart
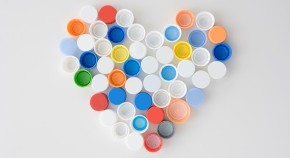
Microplastics at heart of the problem
Patients with carotid artery plaque that contain microplastics and nanoplastics were found to be at higher risk of cardiovascular events and mortality than those in whom the particles were not detected.
- Sonia Muliyil
It may all come down to the mechanisms of nanoparticle delivery
A long-standing nanoparticle delivery paradigm in cancer, that is, the enhanced permeability and retention effect, has been challenged, shifting the focus to active delivery mechanisms, which may provide a new mechanistic foundation for nanoparticle design.
Ultrabright near-infrared light
- Andries Meijerink
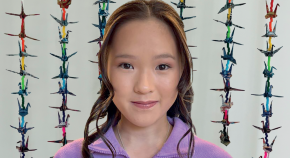
The potential for academics to inspire the next generation
Programmes to inspire the next generation of researchers can have a huge impact, as Rachel Lou explains in the recounting of her own experience.
Quick links
- Explore articles by subject
- Guide to authors
- Editorial policies

Identifiers
Linking ISSN (ISSN-L): 1388-0764
URL http://dx.doi.org/10.1007/11051.1572-896X
Google https://www.google.com/search?q=ISSN+%221572-896X%22
Bing https://www.bing.com/search?q=ISSN+%221572-896X%22
Yahoo https://search.yahoo.com/search?p=ISSN%20%221572-896X%22
Koninklijke Bibliotheek https://webggc.oclc.org/cbs/DB=2.37/SET=4/TTL=1/CMD?ACT=SRCHA&IKT=1007&SRT=RLV&TRM=1572-896X
Pubmed https://pubmed.ncbi.nlm.nih.gov/?term=%221572-896X%22%5BJournal%5D&sort=
Resource information
Archival status.

Title proper: Journal of nanoparticle research.
Country: Netherlands
Medium: Online
Record information
Last modification date: 06/02/2021
Type of record: Confirmed
ISSN Center responsible of the record: ISSN National Centre for The Netherlands
downloads requested
Discover all the features of the complete ISSN records
Display mode x.
Labelled view
MARC21 view
UNIMARC view
- All subject areas
- Agricultural and Biological Sciences
- Arts and Humanities
- Biochemistry, Genetics and Molecular Biology
- Business, Management and Accounting
- Chemical Engineering
- Computer Science
- Decision Sciences
- Earth and Planetary Sciences
- Economics, Econometrics and Finance
- Engineering
- Environmental Science
- Health Professions
- Immunology and Microbiology
- Materials Science
- Mathematics
- Multidisciplinary
- Neuroscience
- Pharmacology, Toxicology and Pharmaceutics
- Physics and Astronomy
- Social Sciences
- All subject categories
- Acoustics and Ultrasonics
- Advanced and Specialized Nursing
- Aerospace Engineering
- Agricultural and Biological Sciences (miscellaneous)
- Agronomy and Crop Science
- Algebra and Number Theory
- Analytical Chemistry
- Anesthesiology and Pain Medicine
- Animal Science and Zoology
- Anthropology
- Applied Mathematics
- Applied Microbiology and Biotechnology
- Applied Psychology
- Aquatic Science
- Archeology (arts and humanities)
- Architecture
- Artificial Intelligence
- Arts and Humanities (miscellaneous)
- Assessment and Diagnosis
- Astronomy and Astrophysics
- Atmospheric Science
- Atomic and Molecular Physics, and Optics
- Automotive Engineering
- Behavioral Neuroscience
- Biochemistry
- Biochemistry, Genetics and Molecular Biology (miscellaneous)
- Biochemistry (medical)
- Bioengineering
- Biological Psychiatry
- Biomaterials
- Biomedical Engineering
- Biotechnology
- Building and Construction
- Business and International Management
- Business, Management and Accounting (miscellaneous)
- Cancer Research
- Cardiology and Cardiovascular Medicine
- Care Planning
- Cell Biology
- Cellular and Molecular Neuroscience
- Ceramics and Composites
- Chemical Engineering (miscellaneous)
- Chemical Health and Safety
- Chemistry (miscellaneous)
- Chiropractics
- Civil and Structural Engineering
- Clinical Biochemistry
- Clinical Psychology
- Cognitive Neuroscience
- Colloid and Surface Chemistry
- Communication
- Community and Home Care
- Complementary and Alternative Medicine
- Complementary and Manual Therapy
- Computational Mathematics
- Computational Mechanics
- Computational Theory and Mathematics
- Computer Graphics and Computer-Aided Design
- Computer Networks and Communications
- Computer Science Applications
- Computer Science (miscellaneous)
- Computer Vision and Pattern Recognition
- Computers in Earth Sciences
- Condensed Matter Physics
- Conservation
- Control and Optimization
- Control and Systems Engineering
- Critical Care and Intensive Care Medicine
- Critical Care Nursing
- Cultural Studies
- Decision Sciences (miscellaneous)
- Dental Assisting
- Dental Hygiene
- Dentistry (miscellaneous)
- Dermatology
- Development
- Developmental and Educational Psychology
- Developmental Biology
- Developmental Neuroscience
- Discrete Mathematics and Combinatorics
- Drug Discovery
- Drug Guides
- Earth and Planetary Sciences (miscellaneous)
- Earth-Surface Processes
- Ecological Modeling
- Ecology, Evolution, Behavior and Systematics
- Economic Geology
- Economics and Econometrics
- Economics, Econometrics and Finance (miscellaneous)
- Electrical and Electronic Engineering
- Electrochemistry
- Electronic, Optical and Magnetic Materials
- Emergency Medical Services
- Emergency Medicine
- Emergency Nursing
- Endocrine and Autonomic Systems
- Endocrinology
- Endocrinology, Diabetes and Metabolism
- Energy Engineering and Power Technology
- Energy (miscellaneous)
- Engineering (miscellaneous)
- Environmental Chemistry
- Environmental Engineering
- Environmental Science (miscellaneous)
- Epidemiology
- Experimental and Cognitive Psychology
- Family Practice
- Filtration and Separation
- Fluid Flow and Transfer Processes
- Food Animals
- Food Science
- Fuel Technology
- Fundamentals and Skills
- Gastroenterology
- Gender Studies
- Genetics (clinical)
- Geochemistry and Petrology
- Geography, Planning and Development
- Geometry and Topology
- Geotechnical Engineering and Engineering Geology
- Geriatrics and Gerontology
- Gerontology
- Global and Planetary Change
- Hardware and Architecture
- Health Informatics
- Health Information Management
- Health Policy
- Health Professions (miscellaneous)
- Health (social science)
- Health, Toxicology and Mutagenesis
- History and Philosophy of Science
- Horticulture
- Human Factors and Ergonomics
- Human-Computer Interaction
- Immunology and Allergy
- Immunology and Microbiology (miscellaneous)
- Industrial and Manufacturing Engineering
- Industrial Relations
- Infectious Diseases
- Information Systems
- Information Systems and Management
- Inorganic Chemistry
- Insect Science
- Instrumentation
- Internal Medicine
- Issues, Ethics and Legal Aspects
- Leadership and Management
- Library and Information Sciences
- Life-span and Life-course Studies
- Linguistics and Language
- Literature and Literary Theory
- LPN and LVN
- Management Information Systems
- Management, Monitoring, Policy and Law
- Management of Technology and Innovation
- Management Science and Operations Research
- Materials Chemistry
- Materials Science (miscellaneous)
- Maternity and Midwifery
- Mathematical Physics
- Mathematics (miscellaneous)
- Mechanical Engineering
- Mechanics of Materials
- Media Technology
- Medical and Surgical Nursing
- Medical Assisting and Transcription
- Medical Laboratory Technology
- Medical Terminology
- Medicine (miscellaneous)
- Metals and Alloys
- Microbiology
- Microbiology (medical)
- Modeling and Simulation
- Molecular Biology
- Molecular Medicine
- Nanoscience and Nanotechnology
- Nature and Landscape Conservation
- Neurology (clinical)
- Neuropsychology and Physiological Psychology
- Neuroscience (miscellaneous)
- Nuclear and High Energy Physics
- Nuclear Energy and Engineering
- Numerical Analysis
- Nurse Assisting
- Nursing (miscellaneous)
- Nutrition and Dietetics
- Obstetrics and Gynecology
- Occupational Therapy
- Ocean Engineering
- Oceanography
- Oncology (nursing)
- Ophthalmology
- Oral Surgery
- Organic Chemistry
- Organizational Behavior and Human Resource Management
- Orthodontics
- Orthopedics and Sports Medicine
- Otorhinolaryngology
- Paleontology
- Parasitology
- Pathology and Forensic Medicine
- Pathophysiology
- Pediatrics, Perinatology and Child Health
- Periodontics
- Pharmaceutical Science
- Pharmacology
- Pharmacology (medical)
- Pharmacology (nursing)
- Pharmacology, Toxicology and Pharmaceutics (miscellaneous)
- Physical and Theoretical Chemistry
- Physical Therapy, Sports Therapy and Rehabilitation
- Physics and Astronomy (miscellaneous)
- Physiology (medical)
- Plant Science
- Political Science and International Relations
- Polymers and Plastics
- Process Chemistry and Technology
- Psychiatry and Mental Health
- Psychology (miscellaneous)
- Public Administration
- Public Health, Environmental and Occupational Health
- Pulmonary and Respiratory Medicine
- Radiological and Ultrasound Technology
- Radiology, Nuclear Medicine and Imaging
- Rehabilitation
- Religious Studies
- Renewable Energy, Sustainability and the Environment
- Reproductive Medicine
- Research and Theory
- Respiratory Care
- Review and Exam Preparation
- Reviews and References (medical)
- Rheumatology
- Safety Research
- Safety, Risk, Reliability and Quality
- Sensory Systems
- Signal Processing
- Small Animals
- Social Psychology
- Social Sciences (miscellaneous)
- Social Work
- Sociology and Political Science
- Soil Science
- Space and Planetary Science
- Spectroscopy
- Speech and Hearing
- Sports Science
- Statistical and Nonlinear Physics
- Statistics and Probability
- Statistics, Probability and Uncertainty
- Strategy and Management
- Stratigraphy
- Structural Biology
- Surfaces and Interfaces
- Surfaces, Coatings and Films
- Theoretical Computer Science
- Tourism, Leisure and Hospitality Management
- Transplantation
- Transportation
- Urban Studies
- Veterinary (miscellaneous)
- Visual Arts and Performing Arts
- Waste Management and Disposal
- Water Science and Technology
- All regions / countries
- Asiatic Region
- Eastern Europe
- Latin America
- Middle East
- Northern America
- Pacific Region
- Western Europe
- ARAB COUNTRIES
- IBEROAMERICA
- NORDIC COUNTRIES
- Afghanistan
- Bosnia and Herzegovina
- Brunei Darussalam
- Czech Republic
- Dominican Republic
- Netherlands
- New Caledonia
- New Zealand
- Papua New Guinea
- Philippines
- Puerto Rico
- Russian Federation
- Saudi Arabia
- South Africa
- South Korea
- Switzerland
- Syrian Arab Republic
- Trinidad and Tobago
- United Arab Emirates
- United Kingdom
- United States
- Vatican City State
- Book Series
- Conferences and Proceedings
- Trade Journals

- Citable Docs. (3years)
- Total Cites (3years)

Follow us on @ScimagoJR Scimago Lab , Copyright 2007-2024. Data Source: Scopus®

Cookie settings
Cookie Policy
Legal Notice
Privacy Policy
- News & Media
- Chemical Biology
- Computational Biology
- Ecosystem Science
- Cancer Biology
- Exposure Science & Pathogen Biology
- Metabolic Inflammatory Diseases
- Advanced Metabolomics
- Mass Spectrometry-Based Measurement Technologies
- Spatial and Single-Cell Proteomics
- Structural Biology
- Biofuels & Bioproducts
- Human Microbiome
- Soil Microbiome
- Synthetic Biology
- Computational Chemistry
- Chemical Separations
- Chemical Physics
- Atmospheric Aerosols
- Human-Earth System Interactions
- Modeling Earth Systems
- Coastal Science
- Plant Science
- Subsurface Science
- Terrestrial Aquatics
- Materials in Extreme Environments
- Precision Materials by Design
- Science of Interfaces
- Friction Stir Welding & Processing
- Dark Matter
- Flavor Physics
- Fusion Energy Science
- Neutrino Physics
- Quantum Information Sciences
- Emergency Response
- AGM Program
- Tools and Capabilities
- Grid Architecture
- Grid Cybersecurity
- Grid Energy Storage
- Earth System Modeling
- Energy System Modeling
- Transmission
- Distribution
- Appliance and Equipment Standards
- Building Energy Codes
- Advanced Building Controls
- Advanced Lighting
- Building-Grid Integration
- Building and Grid Modeling
- Commercial Buildings
- Federal Performance Optimization
- Resilience and Security
- Grid Resilience and Decarbonization
- Building America Solution Center
- Energy Efficient Technology Integration
- Home Energy Score
- Electrochemical Energy Storage
- Flexible Loads and Generation
- Grid Integration, Controls, and Architecture
- Regulation, Policy, and Valuation
- Science Supporting Energy Storage
- Chemical Energy Storage
- Waste Processing
- Radiation Measurement
- Environmental Remediation
- Subsurface Energy Systems
- Carbon Capture
- Carbon Storage
- Carbon Utilization
- Advanced Hydrocarbon Conversion
- Fuel Cycle Research
- Advanced Reactors
- Reactor Operations
- Reactor Licensing
- Solar Energy
- Wind Resource Characterization
- Wildlife and Wind
- Community Values and Ocean Co-Use
- Wind Systems Integration
- Wind Data Management
- Distributed Wind
- Energy Equity & Health
- Environmental Monitoring for Marine Energy
- Marine Biofouling and Corrosion
- Marine Energy Resource Characterization
- Testing for Marine Energy
- The Blue Economy
- Environmental Performance of Hydropower
- Hydropower Cybersecurity and Digitalization
- Hydropower and the Electric Grid
- Materials Science for Hydropower
- Pumped Storage Hydropower
- Water + Hydropower Planning
- Grid Integration of Renewable Energy
- Geothermal Energy
- Algal Biofuels
- Aviation Biofuels
- Waste-to-Energy and Products
- Hydrogen & Fuel Cells
- Emission Control
- Energy-Efficient Mobility Systems
- Lightweight Materials
- Vehicle Electrification
- Vehicle Grid Integration
- Contraband Detection
- Pathogen Science & Detection
- Explosives Detection
- Threat-Agnostic Biodefense
- Discovery and Insight
- Proactive Defense
- Trusted Systems
- Nuclear Material Science
- Radiological & Nuclear Detection
- Nuclear Forensics
- Ultra-Sensitive Nuclear Measurements
- Nuclear Explosion Monitoring
- Global Nuclear & Radiological Security
- Disaster Recovery
- Global Collaborations
- Legislative and Regulatory Analysis
- Technical Training
- Additive Manufacturing
- Deployed Technologies
- Rapid Prototyping
- Systems Engineering
- 5G Security
- RF Signal Detection & Exploitation
- Climate Security
- Internet of Things
- Maritime Security
- Artificial Intelligence
- Graph and Data Analytics
- Software Engineering
- Computational Mathematics & Statistics
- High-Performance Computing
- Visual Analytics
- Lab Objectives
- Publications & Reports
- Featured Research
- Diversity, Equity, Inclusion & Accessibility
- Lab Leadership
- Lab Fellows
- Staff Accomplishments
- Undergraduate Students
- Graduate Students
- Post-graduate Students
- University Faculty
- University Partnerships
- K-12 Educators and Students
- STEM Workforce Development
- STEM Outreach
- Meet the Team
- Internships
- Regional Impact
- Philanthropy
- Volunteering
- Available Technologies
- Industry Partnerships
- Licensing & Technology Transfer
- Entrepreneurial Leave
- Atmospheric Radiation Measurement User Facility
- Electricity Infrastructure Operations Center
- Energy Sciences Center
- Environmental Molecular Sciences Laboratory
- Grid Storage Launchpad
- Institute for Integrated Catalysis
- Interdiction Technology and Integration Laboratory
- PNNL Portland Research Center
- PNNL Seattle Research Center
- PNNL-Sequim (Marine and Coastal Research)
- Radiochemical Processing Laboratory
- Shallow Underground Laboratory
Facet-Dependent Dispersion and Aggregation of Aqueous Hematite Nanoparticles
Published: April 13, 2024
Research topics
Advertisement
Potential and risks of nanotechnology applications in COVID-19-related strategies for pandemic control
- Open access
- Published: 09 November 2023
- Volume 25 , article number 229 , ( 2023 )
Cite this article
You have full access to this open access article
- Fatemeh Araste 1 ,
- Astrid Diana Bakker ORCID: orcid.org/0000-0002-0280-9124 2 &
- Behrouz Zandieh-Doulabi ORCID: orcid.org/0000-0002-0290-3241 2
1475 Accesses
2 Altmetric
Explore all metrics
The ongoing battle against viral infections highlighted so recently by the COVID-19 pandemic demonstrates the need to develop new approaches using nanotechnology in antiviral strategies. Nanoparticles have emerged as promising tools in the fight against viral outbreaks, offering various options for application such as biosensors, vaccine nanoparticles, disinfectants, and functionalized nanoparticles. In this comprehensive review, we evaluate the role of nanoparticles in pandemic control, exploring their potential applications, benefits, and associated risks. We first discuss the importance of nanotechnology in viral outbreak management, particularly in vaccine development. Although lipid nanoparticles play a crucial role in mRNA vaccines, there are concerns about their potential side effects. Although functionalization of protective face masks using metallic nanoparticles has emerged as a sustainable alternative to disposable masks, reducing waste production and enhancing virus filtration, improper disposal of such masks leads to environmental contamination and potential ecological harm. Second, we address the potential adverse effects associated with nanoparticle-based vaccines containing polyethylene glycol and other vaccine components, which trigger autoimmune diseases and alter menstrual cycles. To manage outbreaks effectively, we must minimize such potential risks and environmental impacts. Thus, when developing effective strategies for future pandemic control, it is crucial to understand the advantages and challenges associated with nanoparticle usage.
Similar content being viewed by others
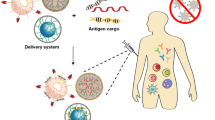
Advances in vaccine delivery systems against viral infectious diseases
Dongyoon Kim, Yina Wu, … Yu-Kyoung Oh
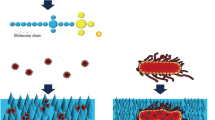
A short review on nanotechnology interventions against COVID-19
Abhimanyu Tharayil, R. Rajakumari, … Nandakumar Kalarikkal
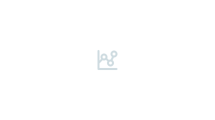
Nanotheranostics and its role in diagnosis, treatment and prevention of COVID-19
Lipsa Leena Panigrahi, Banishree Sahoo & Manoranjan Arakha
Avoid common mistakes on your manuscript.
Introduction
Throughout the twentieth century, viral infections significantly impacted global health, causing millions of deaths worldwide. To combat these diseases, nanotechnology has emerged as a promising approach in the development of antiviral agents such as biosensors, nanoprobes, virus-like particles (VLPs), and functionalized nanoparticles [ 1 , 2 , 3 ]. The COVID-19 pandemic highlighted the importance of nanotechnology in the battle against viral infections, particularly in the development of vaccines. In turn, ongoing discoveries of new virus variants highlight the importance of being prepared to combat potential future pandemics. Recently, for example, three new COVID-related virus variants were discovered in bats in Laos [ 4 ].
In this review, we evaluate the role of nanoparticles in pandemic control and discuss their potential applications, and also the alarms and risks associated with their use. We also draw on the insights learned from the COVID-19 outbreak regarding the use of nanoparticles in managing viral outbreaks. For instance, although antiviral face masks containing metal nanoparticles were proposed as a more sustainable alternative to disposable masks, since they reduce the amount of non-biodegradable waste material [ 5 ], their improper disposal has contributed to the release of metal nanoparticles into the environment. One recent example was their release into Colombian and Southern Brazilian waters [ 6 , 7 ]. Improper disposal may also cause ecological harm [ 8 ]. A comprehensive understanding of the advantages and risks associated with nanoparticle utilization is therefore essential to their responsible and effective use.
Another significant application of nanoparticles in the fight against COVID-19 is the use of lipid nanoparticle platforms for mRNA vaccines. Although the widespread use of lipid nanoparticles containing mRNA vaccines has contributed significantly to pandemic control [ 9 ], the long-term safety and activity of the vaccine-containing nanoparticles are unclear. Neither have the logistics associated with transport — such as special equipment for storage during transport to remote areas, and the costs this involves — been addressed so far.
Below, we explore the various potentials of nanoparticles in viral outbreak management. More specifically, we discuss the use of functionalized face masks for preventing viral infections, the significance of nanoparticle-based drug delivery systems for antiviral therapeutics, and the role of nanoparticles in diagnostic platforms for rapid and accurate viral detection. We also address the concerns and risks associated with the use of nanoparticles, including their potential ecological impacts and other long-term safety considerations. We highlight the critical role of nanoparticles in tackling viral outbreaks, showcasing their potential applications and stressing the importance of their responsible and ethical use. Through proper consideration of the benefits and risks intricately linked with nanoparticle usage, we hope to ensure the development of effective strategies for future pandemic control.
Nanotechnology applications in SARS-CoV-2 transmission prevention
Functionalization of protective face masks using nanoparticles.
The improper disposal of non-reusable face masks has become an environmental concern, as these masks often contain non-biodegradable materials and pathogenic particles in their filter layer. Over time, the mask weathering caused by factors such as mechanical stress, UV-light, or quartz particles causes the release of microplastics from the masks into the environment [ 10 ].
Surgical masks usually consist of three layers, with a central filter layer. To reduce waste production, this filter layer can be functionalized with metallic nanoparticles composed of silver, zinc, or copper, which interfere with viral reproductive cycles, thereby helping to improve virus filtration over that of ordinary masks [ 11 , 12 , 13 , 14 , 15 ]. Metallic nanoparticles can be used to functionalize face masks through the addition of substances such as photo-sensitizing nanoparticles, which produce reactive oxygen species (ROS) upon exposure to specific wavelengths of light, thereby effectively destroying pathogenic membranes, proteins, and nucleic acids after each mask use [ 16 ]. Plasma-based nanoparticles with photo-thermal efficiency, such as graphene, silver, and gold nanoparticles, are able to self-disinfect when exposed to light, and to absorb any moisture present in the mask [ 12 , 17 ]. Graphene-derivatives add other properties to functionalized face masks, such as resistance to smog, mechanical and abrasion stress, and UV light [ 18 ]. Positively charged polymer nanoparticles have strong virucidal properties that reform and fluidize the lipid content of viral membranes, particularly in lipid-raft areas [ 19 ]. Similarly, biodegradable polysaccharide-based materials successfully combat COVID-19 in facial mask layers, achieving complete decomposition in soil within 4 weeks [ 20 , 21 ].
Various nanotechnology applications in SARS-CoV-2 transmission prevention
As well as functionalizing protective face masks, metallic nanoparticles can be applied in mouthwash and nose rinses, offering new opportunities for combating viral infections. As silver nanoparticles (AgNPs) inhibited SARS-CoV-2 in pre-clinical studies [ 22 , 23 ], they may have potential for wider application. Whereas chemical disinfectants need high concentrations of the active substance, metallic nanoparticles can be used in low concentrations, producing less harmful byproducts and being more effective than standard disinfectants [ 24 ]. Another application of nanotechnology involves spraying nano-sized electrostatic atomized water particles (NEAWPs) onto an electrode on which water molecules have first condensed. This significantly reduces the environmental virus count [ 25 ]. Such use of nanoparticles is particularly important because the excessive use of traditional disinfectants during the pandemic increased levels of quaternary ammonium compounds in water and soil, thereby posing an environmental threat [ 26 ].
Nanoparticles as antiviral therapeutic agents
The main antiviral agents against SARS-CoV-2 infection, i.e., remdesivir, zanamivir, oseltamivir, and abacavir, are specific for HIV and/or influenza, but not for SARS-CoV-2 infections [ 27 , 28 ]. The use of nanoparticles in COVID-19 treatment strategies is promising since the nanoparticles do combat SARS-CoV-2. For example, iron oxide nanoparticles interfere with the S1 subunit of the RBD domain [ 29 ], and amyloid-like proteins from LCB1 and LCB3 sequences of the S protein self-assemble into multivalent spherical nanoparticles, competitively blocking viral interaction with the angiotensin-converting enzyme 2 (ACE2) receptor [ 30 ]. Similarly, linear polyglycerol sulfate and its fullerene-conjugated derivative can block virus entry into host cells [ 31 ]. Biological nanovesicles from human lung spheroid cells that present ACE2, as cell-mimicking nanodecoys, are promising, since they absorb viruses and prevent their attachment to the host cells [ 32 ].
Controlled-release systems of antivirals using nanoparticles
Nanoparticles provide a controlled-release system of antivirals to reduce side effects, increase bioavailability, improve circulation time, or ameliorate delivery of the antivirals [ 33 ]. For example, polymeric nanoparticles made of poly-ε-caprolactone (PCL) or poly-lactic glycolic acid-conjugated-poly-ethylene glycol (PLGA-PEG) decorated with ACE2 ligands successfully encapsulate remdesivir, playing dual antiviral roles through competitive interference with SARS-CoV-2 in ACE2 binding, and through targeted drug delivery to lung cells [ 34 ]. The anti-COVID efficacy of the drug is enhanced by PLGA-lipid hybrid nanoparticles encapsulating fluoxetine hydrochloride [ 35 ]. Table 1 provides information on selected types of nanoparticle that are used against different coronaviruses.
Environmental challenges and urgent action
Adverse effects associated with nanoparticles in personal protective equipment.
Despite some positive aspects of metal nanoparticle usage in personal protective equipment (PPEs) such as masks, potentially adverse effects of metal nanoparticles on ecosystems and their fate in the mask-washing process have not been thoroughly investigated. Their entry into the ecosystem may have unintended consequences.
Due to their high surface-to-volume ratio and reactive surface, nanoparticles are prone to interfere with biological processes. While their environmental impact, particularly that of nanoparticles originating from PPEs, has raised concerns about possible adverse effects on the ecosystem, these effects are not entirely negative. As well as aiding the removal of heavy metals and organic pollutants in wastewater treatment, metal nanoparticles can degrade microplastics and nanoplastics, and produce H 2 O and CO 2 as end-products of degradation [ 8 , 36 , 37 ]. Nonetheless, the prolonged and continuous use of nanoparticles in PPEs leads to the accumulation of toxic levels of degradation products, posing risks to various organisms, and to the entire ecosystem [ 38 ]. In aquatic conditions, metal nanoparticles derived from PPEs can interact with other pollutants. The detrimental effects in organisms range from inflammation to cellular damage that further exacerbate any impact on the environment [ 8 ]. A further problem is the improper disposal of PPEs in landfills, dumpsites, marine environments, or public spaces. This can cause animals to mistakenly recognize such PPE waste as food, resulting in their inadvertent and potentially harmful ingestion.
All in all, urgent action is required to address a range of significant environmental challenges. To promote proper disposal practices and prevent the dissemination of nanoparticles into the environment, specific recycling guidelines tailored to nanotechnology products should be established and enforced [ 19 ]. Before the SARS-CoV-2 pandemic, nanoparticles entered the environment mainly through household usage, industrial waste, or laboratory penetration. However, during the initial stages of the pandemic, when it was believed that the virus could be transmitted through surfaces, the use of antiseptic and disinfectant agents skyrocketed, increasing the release of nanoparticles into the environment. Now, in the post-SARS-CoV-2 period, the main source of nanoparticle pollution is the widespread uncontrolled abandonment of personal protective equipment.
Adverse effects of nanoparticles on plants and microorganisms
During the SARS-CoV-2 pandemic, the worldwide demand for masks reached over 4 billion daily, all while recycling programs for masks were inadequately planned [ 39 ]. Several countries used reusable masks containing carbon nanotubes, and/or silver (Ag), silicon dioxide (SiO 2 ), zinc oxide (ZnO), or nanoparticles titanium dioxide (TiO 2 ). Disposal of nanosilver from these masks was found to pose ecological hazards, inhibiting plant growth and photosynthesis [ 40 ]. Engineered nanoparticles commonly penetrate the roots of plants, resulting in phytotoxicity [ 41 ]. As nanoparticles generate reactive ions interacting with nutrients and inorganic compounds in plants, they cause chlorosis and wilting [ 42 , 43 ]. Small-sized nanoparticles such as TiO 2 pass through protective layers like the cuticle, cell wall, and cell membrane [ 44 ], impairing the growth of seedlings crops, the uptake of minerals, and chlorophyll synthesis [ 45 ]. ZnO nanoparticles reduce chlorophyll production in bulb onions, as well as crop growth and development [ 46 , 47 , 48 ]. Ag nanoparticles increase the activity of antioxidant enzymes, reduce chlorophyll content, and impair photosynthesis in tomatoes [ 49 , 50 ].
Similarly adverse effects of nanoparticles are observed not only in plants, but also in bacteria and aquatic animals [ 51 ]. For example, ZnO-based nanoparticles induce genetic mutations in Caenorhabditis elegans , resulting in offspring toxicity [ 52 , 53 ]. In sea water, TiO 2 nanoparticles released from sunscreens cause severe damage to gill filaments, hampering aquatic animal reproduction [ 54 , 55 ]. Overall, it is therefore clear that the adverse effects of nanoparticles eventually disrupt the food chain for higher organisms.
Adverse effects of nanoparticles on higher organisms
The generation of reactive oxygen species is a biological process. Their excessive generation causes oxidative stress, leading to inflammation, diabetes, cancer, and other degenerative diseases [ 56 , 57 ]. Excessive reactive oxygen species causes free-radical production, lipid peroxidation, genotoxicity, and apoptosis [ 58 ]. Nanoparticles accumulate in various organs and have overall systemic effects [ 59 ]. Some inorganic nanoparticles such as TiO 2 , SiO 2 , ZnO, and Fe 2 O 3 dissolve in the acidic environment of the stomach [ 60 ]. Through absorption into the skin, lungs, and liver [ 61 ], they also impair human health.
Toxicity caused by silver nanoparticles (AgNPs) in vitro depends on the surface coating and the concentration of AGNPs it contains. In vivo, AgNPs enter the bloodstream and accumulate in organs, where they cause cytotoxicity [ 62 ]. The generation of Ag + ions from AgNPs and oxidative stress both lead to apoptosis via translocation of mitochondrial cytochrome C into the cytosol [ 63 ], or to necrosis by reducing sulfhydryl groups [ 64 , 65 ]. Table 2 provides an overview of anti-COVID-19-related cytotoxic effects caused by AgNPs and other types of nanoparticles in vitro and in vivo, and the compendial and noncompendial tests used.
Direct contact with titanium dioxide nanoparticles (TiO 2 NPs) affects skin cells in various ways, for example, by impairing their viability, proliferation, and differentiation [ 66 ]. TiO 2 NPs penetrate into the deep layers of the skin are known to be released in sweat [ 67 , 68 , 69 ]. Inhalation of TiO 2 NPs poses a significant health risk: due to the lower protection provided by the olfactory bulb than by the blood–brain barrier, nano-sized materials can penetrate the brain faster through the olfactory nerve than through systemic injection [ 70 , 71 ].
Being in direct contact with the skin and the air we breathe, protective masks containing Ag and TiO 2 nanoparticles are potentially harmful. The presence of these nanoparticles has been shown to significantly inhibit the growth rate of human osteoblasts, indicating that the adverse effects of the masks are not limited to the skin, inhalation, or brain [ 72 ].
The leaching of Ag + or Cu 2+ ions from metal-impregnated masks has also been linked to potential health risks for humans [ 73 ]. Exposure to these nanoparticles through ingestion, inhalation, or dermal penetration can cause toxicity [ 74 ]. Ingestion is followed by exposure to the complex and harsh condition of the gastrointestinal tract, i.e., pH variations, gastric salts, ions, and enzymes. These interactions modify the composition of nanoparticles, leading to biomolecule adsorption and aggregation [ 75 , 76 , 77 ].
Although nanoparticles have toxic effects on the immune system and are involved in oxidative stress-related disorders, many people attribute these disorders to factors such as air pollution. This has led to proposals for public education on proper disposal of personal protective equipment in government-provided trash containers. Long-term monitoring of coastal waste and citizen initiatives for litter collection in populated areas have also been suggested [ 78 , 79 , 80 ], as has the recycling of carbon powders from masks for use in batteries [ 81 ] or renewable fuels [ 82 ], and the promotion of reusable alternatives and cellulose-fiber textiles. Potential disposal methods also include incineration and optimized pyrolysis [ 82 , 83 ].
The toxicity of metal nanoparticles varies according to the size, surficial coating, and shape of the nanoparticles [ 84 ]. The solubility of AgNPs is inversely proportional to the size of the nanoparticle. Due to increased dissolution and cell penetration efficacy, small nanoparticles exhibit high toxicity, with a strong attachment to DNA also causing DNA damage. Use AgNPs sized more than 20 nm shows less genotoxicity [ 85 , 86 ]. As metal nanoparticles sized less than 100 nm cause increased toxicity in vivo, the recommended ranges lie between 100 and 150 nm [ 87 , 88 ].
Toxicity is also influenced by the type of nanoparticle coating. Coating AgNPs with polyvinylpyrrolidone (PVP) has been found to have a greater toxicity and tissue uptake than citrate coatings, while positively charged polymers such as chitosan enhance the toxicity of AgNPs more than citrate-stabilized particles do. A bovine serum albumin (BSA) coating of gold nanoparticles (AuNPs) also leads to greater toxicity and poorer renal clearance than a glutathione (GSH) coating. On the other hand, coating AuNPs with PEG reduces nanoparticle toxicity and appears to be a suitable coating option [ 85 , 89 , 90 , 91 , 92 ].
Nanoparticles in vaccines and risk assessment
The WHO defines vaccines as pharmaceutical formulations that activate the immune system in order to produce specific antibodies, thereby generating protective immunity against a disease caused by a pathogen [ 93 ]. The conventional vaccines developed since the late eighteenth century rely on the discovery of antibodies in patients who have recovered from infections. To elicit an immune response, these use attenuated or inactivated pathogens and purified pathogen fragments [ 94 ]. Second-generation vaccines are produced using recombinant DNA technology in bacteria or in cell cultures [ 95 ].
Recently, a third generation of vaccines has emerged, which introduces the gene encoding the protective antigen into a host cell. By improving antigen processing and its presentation to antigen-presenting cells (APCs), this enhances the activation of CD4 + and CD8 + cells. This recent advance in vaccine technology holds promise for eliciting protective immune responses against the virus [ 96 ].
To overcome the limitations of conventional vaccines, i.e., attenuated or inactivated viruses, alternative options such as RNA- or DNA-based vaccines have also been sought recently. These new RNA- or DNA-based vaccine production technologies aim both to improve reactivity and efficacy and to reduce the cost of vaccines. They can also be used to effectively treat other diseases, such as cancer. But whereas vaccines need to be delivered to the right places in the body in a suitable form so as to prepare the immune system to combat an invading pathogen effectively, most vaccine molecules are prone to degradation. Due to limited accessibility and poor cell permeation [ 97 ], they may not be recognized efficiently by the immune system. A crucial role in enhancing the effectiveness of vaccines is played by delivery systems based on nanoformulations. By tailoring nanoencapsulation, vaccines can be delivered with precision and stability [ 96 ].
These delivery systems contribute to the in vivo behavior of vaccines in various ways: they protect vaccines from enzymatic degradation, improve their pharmacokinetic properties through surface engineering techniques such as PEGylation, enable active targeting to specific organs or cell types, and engineer controlled release of vaccines [ 93 , 98 , 99 ].
Lipid nanoparticles, self-assembling protein nanoparticles, virus-like particles, liposomes, and cationic nanoemulsion vaccines have been designed against SARS-CoV-2 [ 100 ]. The most prominent vaccines against SARS-CoV-2 are lipid-based nanoparticles (LNPs), which have been designed as drug nanocarriers for nucleic acid delivery [ 101 ]. By protecting fragile and unstable nucleic acids from degradation by nucleases, LNPs can increase the half-life of nucleic acids in the blood circulation. Charge-reversible LNPs contain ionizable lipids, either positively or negatively charged, that allow the LNPs to remain neutrally charged in the bloodstream, effective encapsulation of nucleic acids in the LNPs, and a high degree of endosomal escape of LNPs (Fig. 1 ) [ 102 ].
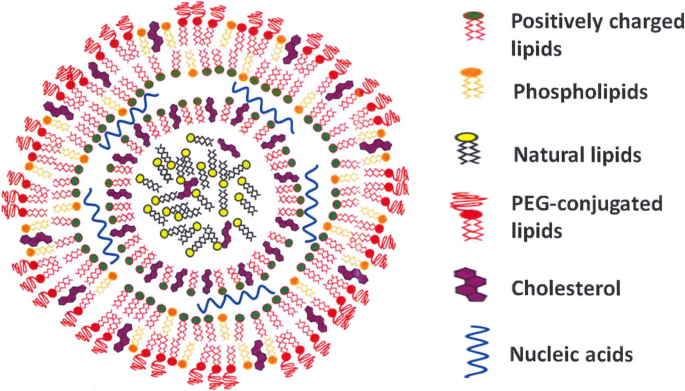
Schematic illustration of an mRNA-based SARS-CoV-2 lipid nanoparticle vaccine. Different components of the vaccine are visualized, i.e., positively charged lipids, phospholipids, natural lipids, PEGylated lipids, cholesterol, and nucleic acids. This figure is modified from those published in [ 105 ]. PEG, polyethylene glycol
LNPs contain two other main components: cholesterol, a neutral phospholipid, and PEG-lipid, which protects LNPs from phagocytosis and aggregation in the blood circulation and also during manufacturing and storage. In vaccine formulations, the PEG-lipid also ensures that the LNP maintains the desired diameter (200 nm) [ 103 ]. A factor that is crucial to efficient nucleic acid delivery is the complete escape of nucleic acids from the endosomal compartment after LNP internalization. By increasing the diffusibility of PEG-lipids, the addition of distearoylphosphatidylcholine (DSPC) and dioleoylphosphatidylethanolamine (DOPE) lipids to nanoparticles enhances endosomal escape [ 104 ].
To produce viral proteins, leading anti-COVID vaccine developers such as Moderna, Pfizer/BioNTech, CureVac, Walvax, Sanofi, Pasteur, and Entos Pharmaceuticals all use cationic LNPs to deliver mRNA or DNA encapsulated into host cells. Although mRNA vaccines are more prone to instability and functional defects than DNA vaccines, they are preferred due to their higher immunogenicity, their direct translation in the cytosol, and their higher loading potential into LNPs [ 106 , 107 , 108 ]. To achieve the same level of efficiency, self-amplifying mRNA-LNP vaccines such as those developed by Imperial College London and Arcturus/Duke-NUS require ten times less mRNA than mRNA vaccines. However, they have less flexibility in nucleotide modification than their mRNA counterparts [ 102 , 109 ].
Most LNP-derived vaccines currently available induce immune responses against the Spike protein (S protein). Interestingly, the receptor binding (RBD) and N-terminal (NTD) domains of the S protein are targeted by the most potent of the 61 monoclonal antibodies isolated from infected patients [ 110 ]. As anti-NTD antibodies inhibit and anti-RBD antibodies neutralize viral infections [ 111 ], the presentation of one of the virus protein domains is preferred above presentation of the whole protein for optimal immunity against new COVID-19 variants.
Due to the need for expensive low-temperature storage required by the SARS-CoV-2 vaccines currently available, their distribution poses challenges in developing countries. As the mechanical stresses caused by shaking might lead to aggregation and mRNA degradation in LNPs, vaccines also need to be administered promptly after preparation [ 112 , 113 , 114 ]. By enhancing the long-term stability of mRNA-LNPs, freeze-drying offers a solution to both these problems. However, if freeze-drying is to be successful, vaccine structure should not be affected by the lyoprotectants and by temperature stress. A new generation of the Pfizer/BioNTech vaccine is currently being prepared in lyophilized (freeze-dried) form [ 115 ].
Adverse effects associated with SARS-CoV-2 nanoparticle vaccines
Mild to moderate side effects are experienced after vaccinations. Compared to conventional vaccines, Pfizer and Moderna vaccines have been shown to cause more serious allergic reactions, including anaphylaxis. While side effects such as flushing and transient dyspnea were also observed in some of these mRNA vaccines, they were not considered to be allergic reactions [ 116 ]. Similar side effects were reported in earlier clinical safety studies of mRNA vaccines against influenza [ 116 ].
Although the rate of allergic reactions for LNP vaccines containing mRNA cargo is generally around 1.31 (95% CI, 0.90–1.84) per million doses, the number of severe immune reactions may be higher with booster doses [ 117 ]. LNPs induce inflammation, especially in non-adherent cells, due to the higher availability of cell surface receptors than in adherent cells [ 118 , 119 ]. The main suspect for anaphylactic reactions in mRNA vaccines is the coating polymer, PEG, which alters the water solubility of the vaccine-containing nanoparticles [ 120 ]. Although PEG is widely used in cosmetics, food, medication, and pharmaceutical agents, its use in vaccine technology is rare [ 121 ].
Initially, PEG molecules were thought to be safe and biologically inert, but nowadays PEG and PEG-like polymers are not considered to be as safe as initially thought [ 122 ]. An immune response mediated by anti-PEG IgG antibodies may develop in allergic individuals, particularly females [ 123 ]. These antibodies can target the PEG backbone or specifically bind to PEG terminal functional groups [ 124 ]. In the presence of reactive oxygen species, anti-PEG antibodies detrimentally affect the respiratory chain and signal transduction pathways, and also disrupt cell membranes [ 125 ]. In vivo, oxidation of PEG, especially of the PEG low-molecular polymer chains, produces toxic molecules, i.e., glycolic acid and hydroxy acid metabolites [ 126 ]. PEGylated nanoparticles cause pseudoallergic reactions such as complement-activation-related pseudo allergy (CARPA) [ 127 ] and toxic or immunogenic responses, particularly with booster doses. Anaphylactic responses to PEG occur in 2–8 cases per year worldwide, which has led the clinical use of two PEGylated pharmaceuticals to be abandoned [ 128 , 129 , 130 ]. The concentration of PEG in mRNA vaccines is much lower than in PEGylated drugs, and intramuscular administration induces less inflammation [ 131 ]. While anaphylactic reactions are caused not only by PEG, allergic reactions are also caused by vaccine components such as polysorbate 80 in the vaccines developed by AstraZeneca and Johnson [ 132 ]. On the other hand, polysorbate 80 is considered to be safer than PEG [ 128 ]. Figure 2 provides a schematic illustration of the various SARS-CoV-2 vaccines, and Table 3 a summary of the side effects associated with them.
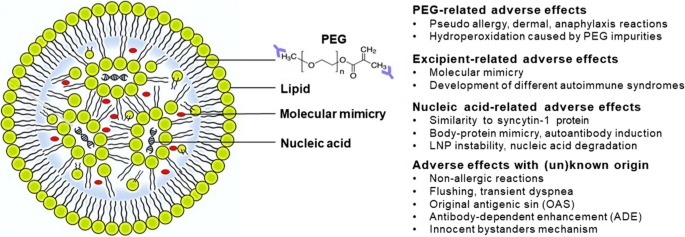
Categorized adverse effects associated with different components of nucleic acid-based lipid nanoparticle vaccines. PEG, excipient, or nucleic-acid-related adverse effects, and adverse effects of known and unknown origin are indicated. Formation of anti-PEG backbone auto-antibodies (blue). PEG, polyethylene glycol
The side effects of PEGylated vaccines and polysorbate-containing vaccines include urticaria, dizziness, diarrhea, wheezing, and tachycardia [ 133 ]. Dermal side effects, such as erythema or swelling, are slightly more common with mRNA vaccines than with adenoviral vaccines (10–15% versus 5–7% of the patients) [ 134 , 135 , 136 ]. Rare side effects of viral vector vaccines include thrombosis and thrombocytopenia. When there is cross-reactivity between PEG and polysorbates, immediate hypersensitivity reactions occur [ 137 , 138 ]. For approximately 6 months, mRNA and adenoviral vaccines can both cause changes in menstrual cycles, such as dysmenorrhea, alterations in frequency, volume, or cessation of bleeding. Women with pre-existing platelet disorders [ 139 ], those taking estrogen-based contraceptives [ 140 ], and those with thrombocytopenia [ 141 ] are all at a higher risk for such changes in their menstrual cycle. Messenger RNA- and viral vaccines affect the menstrual cycle, but the strongest changes are observed with mRNA-LNP vaccines [ 142 , 143 ].
A major concern with the use of nanoparticle vaccines is that they trigger autoimmune diseases. SARS-CoV-2 mRNA-NPs vaccines trigger autoimmune liver diseases, Guillain-Barré syndrome, IgA nephropathy, myocarditis, optical neuromyelitis, autoimmune polyarthritis, Graves’ disease, type 1 diabetes mellitus, and systemic lupus erythematosus [ 144 , 145 , 146 , 147 , 148 , 149 ]. A second concern is the possibility of reverse transcription of mRNA vaccines in liver cells, which has been observed in vitro [ 150 ], although genotoxicity in vivo remains debatable [ 151 ]. A third concern is the phenomenon of original antigenic sin (OAS), which occurs when antibodies from previous infections or vaccinations hinder the neutralization of newly mutated antigens, particularly the omicron antigen variant [ 152 ]. A fourth concern is the concept of antibody-dependent enhancement (ADE), where low antibody titers bind to virus particles without neutralizing them, thereby facilitating virus entry into macrophages and enhancing respiratory disease responses. ADE has been linked to SARS-CoV-2 mRNA-NP vaccines [ 153 ].
A fifth concern has also arisen regarding the cross-reaction of vaccine-induced antibodies against syncytin-1, a placental protein similar to the SARS-CoV-2 spike protein, which activates the immune system and impacts female pregnancy [ 154 ]. Another significant consequence that can arise, mainly in young men, after a second dose of mRNA-based vaccines is myocarditis; this has an incidence of 12.6 cases per million. Sex hormones can contribute to the development of myocarditis [ 155 ], which is not only related to molecular mimicry of S protein, self-antigens, or the formation of autoantibodies, but may also be caused by vaccine adjuvants, activation of “innocent bystanders,” or induction of autoantibodies [ 149 ].
A question we are currently unable to answer is whether the S protein should be replaced by other viral proteins as the immunogenic target to develop vaccines. New generations of vaccines such as spike-trimers and spike-ferritin in liposomes will continue to be based on spike proteins [ 156 , 157 ]. To address and mitigate the side effects of nanoparticle-based vaccines, certain modifications might be considered. PEG chain length and topological configuration affect immunogenicity. Pre-treatment with small amounts of high-molecular-weight PEG reduces anti-PEG reactions, and in animal models, short and hyperbranched PEG polymers, such as poly(oligo-ethylene glycol) methacrylate, exhibit decreased interaction with anti-PEG IgG and IgM antibodies [ 158 ]. To prevent inflammation and side effects, glyceryl monostearate (GMS) should not be included in LNPs [ 118 ]. Promising alternatives for PEG are polyglycerol polyricinoleate, polysarcosine, polyhydroxypropylmethacrylamide, polysulfobetaine, and polycarboxybetaine polymers [ 159 ]. Replacing PEG with polysulfobetaine coating results in higher biological activity of insulin; in nude mice, a dextran coating eliminated toxicity and liver stress of iron oxide nanoparticles [ 160 , 161 ]. Polyesters like polycarbonates and polyphosphoesters might also be viable alternatives to PEG, since they degrade in vivo into non-toxic fragments, and can be easily produced using ring-opening polymerization (ROP) [ 162 ].
More preclinical studies are needed to evaluate vaccines. As two-dimensional in vitro studies may not always completely capture the complex immune environment, and as the phenotype and expression of receptors on cells may be influenced by culture conditions [ 163 ], the prediction of vaccine performance systems might be improved by three-dimensional cell culture systems [ 164 ] and/or standardized in vitro culture systems [ 164 ]. And as small rodents are anatomically different from humans, non-human primates might be more reliable for in vivo studies [ 165 ].
When evaluating nucleic acid vaccines, it is essential to assess not only the quantitative distribution of DNA or mRNA cargo, but also protein expression. This will help to monitor the distribution, retention, and release pattern of the delivered DNA or mRNA, providing a predictive tool for vaccine safety. In addition, valuable insight into the tissue localization of delivered nanoparticles is provided by information on vaccine distribution in lymph nodes, organs, and APCs [ 100 ]. Although a skin-sensitization test is recommended before vaccination with PEG and polysorbate [ 121 , 128 , 166 , 167 ], the number of positive cases in skin tests is considerably lower than the number of sensitive cases after vaccine administration [ 168 ]. Protocols for graded dosing of vaccines have been developed for hypersensitive individuals, such as those with basophil disorders and uncontrolled asthma. Allergic individuals are advised to receive a second dose of a different vaccine, or, in some cases, heterologous prime-boost vaccines are recommended [ 169 , 170 , 171 ]. Finally, in Fig. 3 , we propose several solutions that will minimize the disadvantages associated with nanoparticle use.
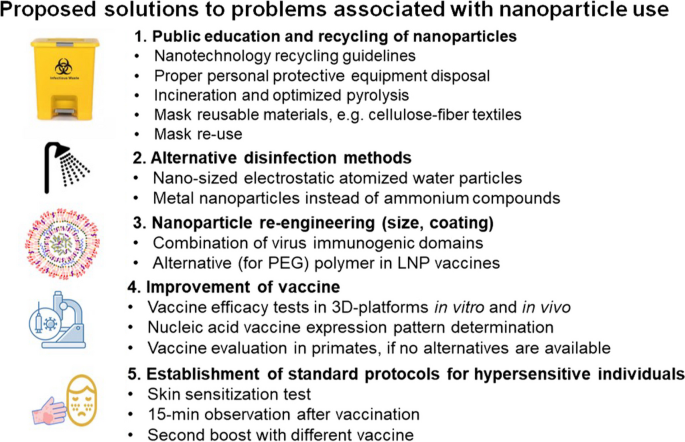
Proposed solutions for minimizing the disadvantages associated with nanoparticle usage
Conclusions
The use of nanoparticles in combatting viral infections has proved to represent a promising and valuable approach in the realm of global health. The COVID-19 pandemic underscored the significance of nanotechnology in vaccine development, infection prevention, and therapeutic strategies. By offering innovative solutions — including functionalized face masks, antiviral therapeutics, and diagnostic platforms — nanoparticles have already showcased their potential in pandemic control. However, the potential risks and challenges associated with their use still require attention, particularly in vaccine development.
Existing LNP formulations have played a crucial role in the rapid development of SARS-CoV-2 vaccines. Unfortunately, vaccine-induced immunity against SARS-CoV-2 is of limited duration, and, to enhance vaccine safety, adverse effects such as anaphylaxis and autoimmune reactions call for modifications in nanoparticle design such as after receiving primary doses, individuals with a history of COVID-19 vaccine anaphylaxis should not receive booster doses of the same vaccine.
The development of long-lasting and immunogenic nanoparticle formulations against SARS-CoV-2 is crucial. To prevent nanoparticle aggregation, surface modification of LNPs is also vital. It is also possible that alternative coating materials, such as shorter-length PEG polymers or other synthetic or natural polymers, may help to minimize side effects and enhance vaccine safety.
Whatever their promise in combating viral infections, the main concerns raised by metal nanoparticles involve their entry into the ecosystem. However, they can also aid in wastewater treatment, microplastic degradation, and environmentally friendly H 2 O and CO 2 production. If responsible nanoparticle use is to be ensured, it is imperative to achieve better control of their toxicity through modifications of nanoparticle size, surface coating, and shape, and also to stimulate public education and proper disposal practices for nanoparticle-based personal protective equipment. Although multiple factors determine whether nanoparticles are toxic, very little information is available on their toxicity, which is sometimes related to the specific drug delivery, and/or to the physical characteristics of the nanoparticles (i.e., their size, surface area, charge, shape, and composition). The adverse effects associated with the use of nanoparticles such as LNPs containing PEG (PEGylated LNPs; Fig. 2 ) limit the use of LNPs in clinical applications. PEG is an FDA-approved compound that is used in pharmacochemical and personal care products. A solution to problems involving its toxicity in clinical uses may be provided by LNP modification, such as by replacing PEG with natural polymers.
The lessons learned from the COVID-19 pandemic have shed light on the importance of responsible and ethical nanoparticle use. In the pursuit of future pandemic control and global health protection, it is essential to continue harnessing the potential of nanotechnology while simultaneously remaining cautious and well-informed. By prioritizing the safety of nanoparticle-based products and vaccines, we will be able to ensure that nanotechnology remains a valuable tool in our fight against viral outbreaks, with minimized risks and enhanced benefits for both human health and the environment.
Kausar S, Said Khan F, Ishaq Mujeeb Ur Rehman M, Akram M, Riaz M, Rasool G, Hamid Khan A, Saleem I, Shamim S, Malik A (2021) A review: mechanism of action of antiviral drugs. Int J Immunopathol Pharmacol 35:731–738. https://doi.org/10.1177/2F20587384211002621
Article Google Scholar
Figueiró Longo JP, Muehlmann LA (2021) How has nanomedical innovation contributed to the COVID-19 vaccine development? Nanomedicine (Lond) 16:731–738. https://doi.org/10.2217/nnm-2021-0035
Article CAS Google Scholar
Chen L, Liang J (2020) An overview of functional nanoparticles as novel emerging antiviral therapeutic agents. Mater Sci Eng C 112:110924. https://doi.org/10.1016/j.msec.2020.110924
Mallapaty S (2021) Laos bats host closest known relatives of virus behind COVID. Nature 597:603. https://doi.org/10.1038/d41586-021-02596-2
Sportelli MC, Izzi M, Kukushkina EA, Hossain SI, Picca RA, Ditaranto N, Cioffi N (2020) Can nanotechnology and materials science help the fight against SARS-CoV-2? Nanomaterials 10:731–738. https://doi.org/10.3390/nano10040802
Silva LF, Dotto GL, Pinto D, Oliveira ML (2021) Nanoparticles and interfaces with toxic elements in fluvial suspended sediment. Mar Pollut Bull 168:112405. https://doi.org/10.1016/j.marpolbul.2021.112405
Oliveira ML, Dotto GL, Pinto D, Neckel A, Silva LF (2021) Nanoparticles as vectors of other contaminants in estuarine suspended sediments: natural and real conditions. Mar Pollut Bull 168:112429. https://doi.org/10.1016/j.marpolbul.2021.112429
López AF, Fabiani M, Lassalle V, Spetter C, Severini MF (2022) Critical review of the characteristics, interactions, and toxicity of micro/nanomaterials pollutants in aquatic environments. Mar Pollut Bull 174:731–738. https://doi.org/10.1016/j.marpolbul.2021.113276
Krantz MS, Phillips EJ (2022) COVID-19 mRNA vaccine safety during the first 6 months of roll-out in the USA. Lancet Infect Dis 35:731–738. https://doi.org/10.1016/S1473-3099(22)00123-2
De-la-Torre GE, Pizarro-Ortega CI, Dioses-Salinas DC, Ammendolia J, Okoffo ED (2021) Investigating the current status of COVID-19 related plastics and their potential impact on human health. Curr Opin Toxicol 35:731–738. https://doi.org/10.1016/j.cotox.2021.08.002
Campos EV, Pereira AE, De Oliveira JL, Carvalho LB, Guilger-Casagrande M, De Lima R, Fraceto LF (2020) How can nanotechnology help to combat COVID-19? Opportunities and urgent need. J Nanobiotechnology 18:1–23. https://doi.org/10.1186/s12951-020-00685-4
Seidi F, Deng C, Zhong Y, Liu Y, Huang Y, Li C, Xiao H (2021) Functionalized masks: powerful materials against COVID-19 and future pandemics. Small 17:2102453. https://doi.org/10.1002/smll.202102453
Bradley D (2020) Copper against COVID. Mater Today (Kidlington, England) 40:2. https://doi.org/10.1016/j.mattod.2020.09.016
Skalny AV, Rink L, Ajsuvakova OP, Aschner M, Gritsenko VA, Alekseenko SI, Svistunov AA, Petrakis D, Spandidos DA, Aaseth J (2020) Zinc and respiratory tract infections: perspectives for COVID-19. Int J Mol Med 46:17–26. https://doi.org/10.3892/ijmm.2020.4575
Sousa BC, Cote DL (2020) Antimicrobial copper cold spray coatings and SARS-CoV-2 surface inactivation. MRS Adv 5:2873–2880. https://doi.org/10.1557/adv.2020.366
Joost U, Juganson K, Visnapuu M, Mortimer M, Kahru A, Nõmmiste E, Kisand V, Ivask A (2015) Photocatalytic antibacterial activity of nano-TiO 2 (anatase)-based thin films: effects on Escherichia coli cells and fatty acids. J Photochem Photobiol B Biol 142:178–185. https://doi.org/10.1016/j.jphotobiol.2014.12.010
Xiao MF, Zeng C, Li SH, Yuan FL (2021) Applications of nanomaterials in COVID-19 pandemic. Rare Met 41:1–13. https://doi.org/10.1007/s12598-021-01789-y
Bhattacharjee S, Joshi R, Chughtai AA, Macintyre CR (2019) Graphene modified multifunctional personal protective clothing. Adv Mater Interfaces 6:1900622. https://doi.org/10.1002/admi.201900622
Palmieri V, De Maio F, De Spirito M, Papi M (2021) Face masks and nanotechnology: keep the blue side up. Nano Today 37:101077. https://doi.org/10.1016/j.nantod.2021.101077
El-Atab N, Qaiser N, Badghaish H, Shaikh SF, Hussain MM (2020) Flexible nanoporous template for the design and development of reusable anti-COVID-19 hydrophobic face masks. ACS Nano 14:7659–7665. https://doi.org/10.1021/acsnano.0c03976
Choi S, Jeon H, Jang M, Kim H, Shin G, Koo JM, Lee M, Sung HK, Eom Y, Yang HS (2021) Biodegradable, efficient, and breathable multi-use face mask filter. Adv Sci 8:2003155. https://doi.org/10.1002/advs.202003155
Almanza-Reyes H, Moreno S, Plascencia-López I, Alvarado-Vera M, Patrón-Romero L, Borrego B, Reyes-Escamilla A, Valencia-Manzo D, Brun A, Pestryakov A (2021) Evaluation of silver nanoparticles for the prevention of SARS-CoV-2 infection in health workers: in vitro and in vivo. Plos One 16:e0256401. https://doi.org/10.1371/journal.pone.0256401
ClinicalTrials.gov (2021) Evaluation of silver nanoparticles for the prevention of COVID-19 (COVID-19). (NCT04894409). https://clinicaltrials.gov/ct2/show/NCT04894409
Talebian S, Wallace GG, Schroeder A, Stellacci F, Conde J (2020) Nanotechnology-based disinfectants and sensors for SARS-CoV-2. Nat Nanotechnol 15:618–621. https://doi.org/10.1038/s41565-020-0751-0
Yasugi M, Komura Y, Ishigami Y (2022) Mechanisms underlying inactivation of SARS-CoV-2 by nano-sized electrostatic atomized water particles. J Nanopart Res 24:99. https://doi.org/10.1007/s11051-022-05485-5
Hora PI, Pati SG, McNamara PJ, Arnold WA (2020) Increased use of quaternary ammonium compounds during the SARS-CoV-2 pandemic and beyond: consideration of environmental implications. Environ Sci Technol Lett 7:622–631. https://doi.org/10.1021/acs.estlett.0c00437
Riva L, Yuan S, Yin X, Martin-Sancho L, Matsunaga N, Pache L, Burgstaller-Muehlbacher S, De Jesus PD, Teriete P, Hull MV (2020) Discovery of SARS-CoV-2 antiviral drugs through large-scale compound repurposing. Nature 586:113–119. https://doi.org/10.1038/s41586-020-2577-1
De Clercq E (2006) Antiviral agents active against influenza A viruses. Nat Rev Drug Discov 5:1015–1025. https://doi.org/10.1038/nrd2175
Abo-Zeid Y, Ismail NS, McLean GR, Hamdy NM (2020) A molecular docking study repurposes FDA approved iron oxide nanoparticles to treat and control COVID-19 infection. Eur J Pharm Sci 153:105465. https://doi.org/10.1016/j.ejps.2020.105465
Behbahanipour M, Benoit R, Navarro S, Ventura S (2023) Oligobinders: bioengineered soluble amyloid-like nanoparticles to bind and neutralize SARS-CoV-2. ACS Appl Mater Interfaces 15:11444–11457. https://doi.org/10.1021/acsami.2c18305
Page TM, Nie C, Neander L, Povolotsky TL, Sahoo AK, Nickl P, Adler JM, Bawadkji O, Radnik J, Achazi K (2023) Functionalized fullerene for inhibition of SARS-CoV-2 variants. Small 19:2206154. https://doi.org/10.1002/smll.202206154
Li Z, Wang Z, Dinh P-UC, Zhu D, Popowski KD, Lutz H, Hu S, Lewis MG, Cook A, Andersen H (2021) Cell-mimicking nanodecoys neutralize SARS-CoV-2 and mitigate lung injury in a non-human primate model of COVID-19. Nat Nanotechnol 16:942–951. https://doi.org/10.1038/s41565-021-00923-2
Milovanovic M, Arsenijevic A, Milovanovic J, Kanjevac T, Arsenijevic N (2017) Nanoparticles in antiviral therapy. In: Antimicrobial nanoarchitectonics. Elsevier, pp 383–410. https://doi.org/10.1016/B978-0-323-52733-0.00014-8
Sanna V, Satta S, Hsiai T, Sechi M (2022) Development of targeted nanoparticles loaded with antiviral drugs for SARS-CoV-2 inhibition. Eur J Med Chem 231:114121. https://doi.org/10.1016/j.ejmech.2022.114121
Khater SE, El-Khouly A, Abdel-Bar HM, Al-Mahallawi AM, Ghorab DM (2021) Fluoxetine hydrochloride loaded lipid polymer hybrid nanoparticles showed possible efficiency against SARS-CoV-2 infection. Int J Pharm 607:121023. https://doi.org/10.1016/j.ijpharm.2021.121023
Naseem T, Durrani T (2021) The role of some important metal oxide nanoparticles for wastewater and antibacterial applications: a review. Environ Chem Ecotoxicol 3:59–75. https://doi.org/10.1016/j.enceco.2020.12.001
Uheida A, Mejía HG, Abdel-Rehim M, Hamd W, Dutta J (2021) Visible light photocatalytic degradation of polypropylene microplastics in a continuous water flow system. J Hazard Mater 406:124299. https://doi.org/10.1016/j.jhazmat.2020.124299
Hydzik P (2012) Nanoparticles toxicity--selective examples. Przegl Lek 69:486–489. PMID: 23243914 https://europepmc.org/article/med/23243914
Shirvanimoghaddam K, Czech B, Yadav R, Gokce C, Fusco L, Delogu LG, Yilmazer A, Brodie G, Al-Othman AK, Al-Tamimi AK (2022) Facemask global challenges: the case of effective synthesis, utilization, and environmental sustainability. Sustainability 14:737. https://doi.org/10.3390/su14020737
Vishwakarma K, Upadhyay N, Singh J, Liu S, Singh VP, Prasad SM, Chauhan DK, Tripathi DK, Sharma S (2017) Differential phytotoxic impact of plant-mediated silver nanoparticles (AgNPs) and silver nitrate (AgNO3) on Brassica sp. Front Plant Sci 8:1501. https://doi.org/10.1016/j.aquatox.2016.04.019
Dietz K-J, Herth S (2011) Plant nanotoxicology. Trends Plant Sci 16:582–589. https://doi.org/10.1016/j.tplants.2011.08.003
Begum P, Fugetsu B (2012) Phytotoxicity of multi-walled carbon nanotubes on red spinach (Amaranthus tricolor L) and the role of ascorbic acid as an antioxidant. J Hazard Mater 243:212–222. https://doi.org/10.1016/j.jhazmat.2012.10.025
Slomberg DL, Schoenfisch MH (2012) Silica nanoparticle phytotoxicity to Arabidopsis thaliana. Environ Sci Technol 46:10247–10254. https://doi.org/10.1021/es300949f
Khan M, Khan MSA, Borah KK, Goswami Y, Hakeem KR, Chakrabartty I (2021) The potential exposure and hazards of metal-based nanoparticles on plants and environment, with special emphasis on ZnO NPs, TiO 2 NPs, and AgNPs: a review. Environ Adv 6:100128. https://doi.org/10.1016/j.envadv.2021.100128
Dağhan H (2018) Effects of TiO2 nanoparticles on maize (Zea mays L.) growth, chlorophyll content, and nutrient uptake. Appl Ecol Environ Res 16:6873–6883. https://doi.org/10.15666/aeer/1605_68736883
Lin D, Xing B (2008) Root uptake and phytotoxicity of ZnO nanoparticles. Environ Sci Technol 42:5580–5585. https://doi.org/10.1021/es800422x
Monica RC, Cremonini R (2009) Nanoparticles and higher plants. Caryologia 62:161–165. https://doi.org/10.1080/00087114.2004.10589681
Raskar S, Laware S (2014) Effect of zinc oxide nanoparticles on cytology and seed germination in onion. Int J Curr Microbiol App Sci 3:467–473. https://www.ijcmas.com/vol-3-2/S.V.Raskar%20and%20S.L.Laware.pdf
Li CC, Wang YJ, Dang F, Zhou DM (2016) Mechanistic understanding of reduced AgNP phytotoxicity induced by extracellular polymeric substances. J Hazard Mater 308:21–28. https://doi.org/10.1016/j.jhazmat.2016.01.036
Wang C, Jiang K, Wu B, Zhou J, Lv Y (2018) Silver nanoparticles with different particle sizes enhance the allelopathic effects of Canada goldenrod on the seed germination and seedling development of lettuce. Ecotoxicology 27:1116–1125. https://doi.org/10.1007/s10646-018-1966-9
Federici G, Shaw BJ, Handy RD (2007) Toxicity of titanium dioxide nanoparticles to rainbow trout (Oncorhynchus mykiss): gill injury, oxidative stress, and other physiological effects. Aquat Toxicol 84:415–430. https://doi.org/10.1016/j.aquatox.2007.07.009
Huang CW, Li SW, Liao VH-C (2017) Chronic ZnO-NPs exposure at environmentally relevant concentrations results in metabolic and locomotive toxicities in Caenorhabditis elegans. Environ Pollut 220:1456–1464. https://doi.org/10.1016/j.envpol.2016.10.086
Bundschuh M, Filser J, Lüderwald S, McKee MS, Metreveli G, Schaumann GE, Schulz R, Wagner S (2018) Nanoparticles in the environment: where do we come from, where do we go to? Environ Sci Europe 30:1–17. https://doi.org/10.1186/s12302-018-0132-6
Labille J, Slomberg D, Catalano R, Robert S, Apers-Tremelo M-L, Boudenne J-L, Manasfi T, Radakovitch O (2020) Assessing UV filter inputs into beach waters during recreational activity: a field study of three French Mediterranean beaches from consumer survey to water analysis. Sci Total Environ 706:136010. https://doi.org/10.1016/j.scitotenv.2019.136010
Wang J, Zhu X, Zhang X, Zhao Z, Liu H, George R, Wilson-Rawls J, Chang Y, Chen Y (2011) Disruption of zebrafish (Danio rerio) reproduction upon chronic exposure to TiO 2 nanoparticles. Chemosphere 83:461–467. https://doi.org/10.1016/j.chemosphere.2010.12.069
Bodamyali T, Stevens CR, Blake DR, Winyard PG (2000) Reactive oxygen/nitrogen species and acute inflammation: a physiological process. In: Free Radicals and Inflammation. Springer, pp 11–16. https://doi.org/10.1007/978-3-0348-8482-2_2
Jakubczyk K, Dec K, Kałduńska J, Kawczuga D, Kochman J, Janda K (2020) Reactive oxygen species-sources, functions, oxidative damage. Pol Merkur Lekarski 48:124–127
Google Scholar
Valko M, Leibfritz D, Moncol J, Cronin MTD, Mazur M, Telser J (2007) Free radicals and antioxidants in normal physiological functions and human disease. Int J Biochem Cell Biol 39:44–84. https://doi.org/10.1016/j.biocel.2006.07.001
Natarajan P, Tomich JM (2020) Understanding the influence of experimental factors on bio-interactions of nanoparticles: towards improving correlation between in vitro and in vivo studies. Arch Biochem Biophys 694:108592. https://doi.org/10.1016/j.abb.2020.108592
Sohal IS, Cho YK, O’Fallon KS, Gaines P, Demokritou P, Bello D (2018) Dissolution behavior and biodurability of ingested engineered nanomaterials in the gastrointestinal environment. ACS Nano 12:8115–8128. https://doi.org/10.1021/acsnano.8b02978
De Matteis V (2017) Exposure to inorganic nanoparticles: routes of entry, immune response, biodistribution and in vitro/in vivo toxicity evaluation. Toxics 5:29. https://doi.org/10.3390/toxics5040029
Yang L, Ji W, Mao M, Huang J-n (2020) An updated review on the properties, fabrication and application of hybrid-nanofluids along with their environmental effects. J Clean Prod 257:120408. https://doi.org/10.1016/j.jclepro.2020.120408
Hsin Y-H, Chen C-F, Huang S, Shih T-S, Lai P-S, Chueh PJ (2008) The apoptotic effect of nanosilver is mediated by a ROS-and JNK-dependent mechanism involving the mitochondrial pathway in NIH3T3 cells. Toxicol Lett 179:130–139. https://doi.org/10.1016/j.toxlet.2008.04.015
ATSDR (2014) Agency for Toxic Substances and Disease Registry (ATSDR), Toxicological profile for silver. https://wwwn.cdc.gov/TSP/ToxProfiles/ToxProfiles.aspx?id=539&tid=97 . Accessed 26 March 2014
Rezvani E, Rafferty A, McGuinness C, Kennedy J (2019) Adverse effects of nanosilver on human health and the environment. Acta Biomater 94:145–159. https://doi.org/10.1016/j.actbio.2019.05.042
Kiss B, Bíró T, Czifra G, Tóth BI, Kertész Z, Szikszai Z, Kiss ÁZ, Juhász I, Zouboulis CC, Hunyadi J (2008) Investigation of micronized titanium dioxide penetration in human skin xenografts and its effect on cellular functions of human skin-derived cells. Exp Dermatol 17:659–667. https://doi.org/10.1111/j.1600-0625.2007.00683.x
Lademann J, Weigmann H-J, Rickmeyer C, Barthelmes H, Schaefer H, Mueller G, Sterry W (1999) Penetration of titanium dioxide microparticles in a sunscreen formulation into the horny layer and the follicular orifice. Skin Pharmacol Physiol 12:247–256. https://doi.org/10.1159/000066249
Bennat C, Müller-Goymann C (2000) Skin penetration and stabilization of formulations containing microfine titanium dioxide as physical UV filter. Int J Cosmet Sci 22:271–283. https://doi.org/10.1046/j.1467-2494.2000.00009.x
Mavon A, Miquel C, Lejeune O, Payre B, Moretto P (2007) In vitro percutaneous absorption and in vivo stratum corneum distribution of an organic and a mineral sunscreen. Skin Pharmacol Physiol 20:10–20. https://doi.org/10.1159/000096167
Christensen FM, Johnston HJ, Stone V, Aitken RJ, Hankin S, Peters S, Aschberger K (2011) Nano-TiO 2 –feasibility and challenges for human health risk assessment based on open literature. Nanotoxicology 5:110–124. https://doi.org/10.3109/17435390.2010.504899
Oberdörster G, Sharp Z, Atudorei V, Elder A, Gelein R, Kreyling W, Cox C (2004) Translocation of inhaled ultrafine particles to the brain. Inhal Toxicol 16:437–445. https://doi.org/10.1080/08958370490439597
Gutwein LG, Webster TJ (2004) Increased viable osteoblast density in the presence of nanophase compared to conventional alumina and titania particles. Biomaterials 25:4175–4183. https://doi.org/10.1016/j.biomaterials.2003.10.090
Pollard ZA, Karod M, Goldfarb JL (2021) Metal leaching from antimicrobial cloth face masks intended to slow the spread of COVID-19. Sci Rep 11:19216. https://doi.org/10.1038/s41598-021-98577-6
Mercer RR, Scabilloni JF, Wang L, Battelli LA, Antonini JM, Roberts JR, Qian Y, Sisler JD, Castranova V, Porter DW (2018) The fate of inhaled nanoparticles: detection and measurement by enhanced dark-field microscopy. Toxicol Pathol 46:28–46. https://doi.org/10.1177/0192623317732321
Coreas R, Cao X, DeLoid GM, Demokritou P, Zhong W (2020) Lipid and protein corona of food-grade TiO2 nanoparticles in simulated gastrointestinal digestion. NanoImpact 20:100272. https://doi.org/10.1016/j.impact.2020.100272
Baimanov D, Wang J, Zhang J, Liu K, Cong Y, Shi X, Zhang X, Li Y, Li X, Qiao R (2022) In situ analysis of nanoparticle soft corona and dynamic evolution. Nat Commun 13:5389. https://doi.org/10.1038/s41467-022-33044-y
Zhou H, McClements DJ (2022) Recent advances in the gastrointestinal fate of organic and inorganic nanoparticles in foods. Nanomaterials 12:1099. https://doi.org/10.3390/nano12071099
Li L, Huang J, Almutairi AW, Lan X, Zheng L, Lin Y, Chen L, Fu N, Lin Z, Abomohra AE-F (2021) Integrated approach for enhanced bio-oil recovery from disposed face masks through co-hydrothermal liquefaction with Spirulina platensis grown in wastewater. Biomass Conv Bioref 13:11109–11120. https://doi.org/10.1007/s13399-021-01891-2
Li S, Ding J, Zheng X, Sui Y (2021) Beach tourists behavior and beach management strategy under the ongoing prevention and control of the COVID-19 pandemic: a case study of Qingdao. China Ocean Coast Manage 215:105974. https://doi.org/10.1016/j.ocecoaman.2021.105974
Hatami T, Rakib MRJ, Madadi R, De-la-Torre GE, Idris AM (2022) Personal protective equipment (PPE) pollution in the Caspian Sea, the largest enclosed inland water body in the world. Sci Total Environ 824:153771. https://doi.org/10.1016/j.scitotenv.2022.153771
Lee G, Lee ME, Kim SS, Joh H-I, Lee S (2022) Efficient upcycling of polypropylene-based waste disposable masks into hard carbons for anodes in sodium ion batteries. J Ind Eng Chem 105:268–277. https://doi.org/10.1016/j.jiec.2021.09.026
Zhao X, Klemeš JJ, You F (2022) Energy and environmental sustainability of waste personal protective equipment (PPE) treatment under COVID-19. Renew Sustain Energy Rev 153:111786. https://doi.org/10.1016/j.rser.2021.111786
Aragaw TA, Mekonnen BA (2021) Current plastics pollution threats due to COVID-19 and its possible mitigation techniques: a waste-to-energy conversion via pyrolysis. Environ Syst Res 10. https://doi.org/10.1186/s40068-020-00217-x
Sani A, Cao C, Cui D (2021) Toxicity of gold nanoparticles (AuNPs): a review. Biochem Biophys Rep 26:100991. https://doi.org/10.1016/j.bbrep.2021.100991
Kim K-T, Truong L, Wehmas L, Tanguay RL (2013) Silver nanoparticle toxicity in the embryonic zebrafish is governed by particle dispersion and ionic environment. Nanotechnology 24:115101. https://doi.org/10.1093/toxsci/kft081
Park MV, Neigh AM, Vermeulen JP, de la Fonteyne LJ, Verharen HW, Briedé JJ, van Loveren H, de Jong WH (2011) The effect of particle size on the cytotoxicity, inflammation, developmental toxicity, and genotoxicity of silver nanoparticles. Biomaterials 32:9810–9817. https://doi.org/10.1016/j.biomaterials.2011.08.085
Renwick L, Brown D, Clouter A, Donaldson K (2004) Increased inflammation and altered macrophage chemotactic responses caused by two ultrafine particle types. Occup Environ Med 61:442–447. https://doi.org/10.1136/oem.2003.008227
Sager TM, Kommineni C, Castranova V (2008) Pulmonary response to intratracheal instillation of ultrafine versus fine titanium dioxide: role of particle surface area. Part Fibre Toxicol 5:17. https://doi.org/10.1186/1743-8977-5-17
Hunt PR, Keltner Z, Gao X, Oldenburg SJ, Bushana P, Olejnik N, Sprando RL (2014) Bioactivity of nanosilver in Caenorhabditis elegans: effects of size, coat, and shape. Toxicol Rep 1:923–944. https://doi.org/10.1016/j.toxrep.2014.10.020
Nativo P, Prior IA, Brust M (2008) Uptake and intracellular fate of surface-modified gold nanoparticles. ACS Nano 2:1639–1644. https://doi.org/10.1021/nn800330a
Cruje C, Chithrani B (2015) Integration of peptides for enhanced uptake of pegylated gold nanoparticles. J Nanosci Nanotechnol 15:2125–2131. https://doi.org/10.1166/jnn.2015.10321
Zhang X-D, Wu D, Shen X, Liu P-X, Fan F-Y, Fan S-J (2012) In vivo renal clearance, biodistribution, toxicity of gold nanoclusters. Biomaterials 33:4628–4638. https://doi.org/10.1016/j.biomaterials.2012.03.020
Pollard AJ, Bijker EM (2021) A guide to vaccinology: from basic principles to new developments. Nat Rev Immunol 21:83–100. https://doi.org/10.1038/s41577-020-00497-5
Riedel S (2005) Edward Jenner and the history of smallpox and vaccination. Baylor University Med Center Proc 2005. Taylor & Francis. https://doi.org/10.1080/08998280.2005.11928028
Nascimento I, Leite L (2012) Recombinant vaccines and the development of new vaccine strategies. Braz J Med Biol Res 45:1102–1111. https://pubmed.ncbi.nlm.nih.gov/22948379/ , https://doi.org/10.1590/s0100-879x2012007500142
Lozano D, Larraga V, Vallet-Regí M, Manzano M (2023) An overview of the use of nanoparticles in vaccine development. Nanomaterials 13:1828. https://doi.org/10.3390/nano13121828
Delany I, Rappuoli R, De Gregorio E (2014) Vaccines for the 21st century. EMBO Mol Med 6:708–720. https://doi.org/10.1002/emmm.201403876
Kirtane AR, Verma M, Karandikar P, Furin J, Langer R, Traverso G (2021) Nanotechnology approaches for global infectious diseases. Nat Nanotechnol 16:369–384. https://doi.org/10.1038/s41565-021-00866-8
Gregory AE, Titball R, Williamson D (2013) Vaccine delivery using nanoparticles. Front Cell Infect Microbiol 3:13. https://doi.org/10.3389/fcimb.2013.00013
Lee J, Kim D, Byun J, Wu Y, Park J, Oh Y-K (2022) In vivo fate and intracellular trafficking of vaccine delivery systems. Adv Drug Deliv Rev 186:114325. https://doi.org/10.1016/j.addr.2022.114325
Akinc A, Maier MA, Manoharan M, Fitzgerald K, Jayaraman M, Barros S, Ansell S, Du X, Hope MJ, Madden TD (2019) The Onpattro story and the clinical translation of nanomedicines containing nucleic acid-based drugs. Nat Nanotechnol 14:1084–1087. https://doi.org/10.1038/s41565-019-0591-y
Buschmann MD, Carrasco MJ, Alishetty S, Paige M, Alameh MG, Weissman D (2021) Nanomaterial delivery systems for mRNA vaccines. Vaccines 9:65. https://doi.org/10.3390/vaccines9010065
Evers MJ, Kulkarni JA, van der Meel R, Cullis PR, Vader P, Schiffelers RM (2018) State-of-the-art design and rapid-mixing production techniques of lipid nanoparticles for nucleic acid delivery. Small Methods 2:1700375. https://doi.org/10.1002/smtd.201700375
Hou X, Zaks T, Langer R, Dong Y (2021) Lipid nanoparticles for mRNA delivery. Nat Rev Mater 7:65. https://doi.org/10.1038/s41578-021-00400-1
Kulkarni JA, Witzigmann D, Thomson SB, Chen S, Leavitt BR, Cullis PR, van der Meel R (2020) The current landscape of nucleic acid therapeutics. Nat Nanotechnol 15:963–963. https://doi.org/10.1038/s41565-021-00898-0
Klauer AA, van Hoof A (2012) Degradation of mRNAs that lack a stop codon: a decade of nonstop progress. Wiley Interdiscip Rev RNA 3:649–660. https://doi.org/10.1002/wrna.1124
Pardi N, Hogan MJ, Porter FW, Weissman D (2018) mRNA vaccines - a new era in vaccinology. Nat Rev Drug Discov 17:261–279. https://doi.org/10.1038/nrd.2017.243
Kulkarni JA, Witzigmann D, Thomson SB, Chen S, Leavitt BR, Cullis PR, van der Meel R (2021) The current landscape of nucleic acid therapeutics. Nat Nanotechnol 16:630–643. https://doi.org/10.1038/s41565-021-00898-0
Bloom K, van den Berg F, Arbuthnot P (2021) Self-amplifying RNA vaccines for infectious diseases. 28:117–129. https://doi.org/10.1038/s41434-020-00204-y
Liu L, Wang P, Nair MS, Yu J, Rapp M, Wang Q, Luo Y, Chan JF-W, Sahi V, Figueroa A (2020) Potent neutralizing antibodies against multiple epitopes on SARS-CoV-2 spike. Nature 584:450–456. https://doi.org/10.1038/s41586-020-2571-7
Gamelin FX, Baquet G, Berthoin S, Thevenet D, Nourry C, Nottin S, Bosquet L (2021) Scientific rationale for developing potent RBD-based vaccines targeting COVID-19. npj Vaccines 6:128. https://doi.org/10.1038/s41541-021-00393-6
European Medicines Agency (EMA). (2021). Comirnaty EPAR public assessment report. CHMP Committee for Medicinal Products for Human Use. https://www.ema.europa.eu/en/documents/assessment-report/comirnaty-epar-public-assessment-report_en.pdf . Accessed 19 February 2021
Selmin F, Musazzi UM, Franzè S, Scarpa E, Rizzello L, Procacci P, Minghetti P (2021) Pre-drawn syringes of Comirnaty for an efficient COVID-19 mass vaccination: demonstration of stability. Pharmaceutics 13:1029. https://doi.org/10.3390/pharmaceutics13071029
Gruber MF (2020) Emergency use authorization (EUA) for an unapproved product review memorandum, in FDA.gov. https://www.fda.gov/media/144416/download . Accessed 20 November 2020
Dolgin E (2020) COVID-19 vaccines poised for launch, but impact on pandemic unclear. Nat Biotechnol. https://doi.org/10.1038/d41587-020-00022-y.Accessed25November2020
Bahl K, Senn JJ, Yuzhakov O, Bulychev A, Brito LA, Hassett KJ, Laska ME, Smith M, Almarsson Ö, Thompson J (2017) Preclinical and clinical demonstration of immunogenicity by mRNA vaccines against H10N8 and H7N9 influenza viruses. Mol Ther 25:1316–1327. https://doi.org/10.1016/j.ymthe.2017.03.035
Reuben RC, Adogo LY (2021) SARS-CoV-2 vaccines–induced thrombotic thrombocytopenia: should we consider immuno-hypersensitivity? Rev Saude Publica 55:70. https://doi.org/10.11606/s1518-8787.2021055003855
Winter E, Pizzol CD, Locatelli C, Crezkynski-Pasa TB (2016) Development and evaluation of lipid nanoparticles for drug delivery: study of toxicity in vitro and in vivo. J Nanosci Nanotechnol 16:1321–1330. https://doi.org/10.1166/jnn.2016.11667
Ng KK, Lovell JF, Zheng G (2011) Lipoprotein-inspired nanoparticles for cancer theranostics. Acc Chem Res 44:1105–1113. https://doi.org/10.1021/ar200017e
Sellaturay P, Nasser S, Islam S, Gurugama P, Ewan PW (2021) Polyethylene glycol (PEG) is a cause of anaphylaxis to the Pfizer/BioNTech mRNA COVID-19 vaccine. Clin Exp Allergy 51:861. https://doi.org/10.1111/cea.13874
Banerji A, Wickner PG, Saff R, Stone CA Jr, Robinson LB, Long AA, Wolfson AR, Williams P, Khan DA, Phillips E (2021) mRNA vaccines to prevent COVID-19 disease and reported allergic reactions: current evidence and suggested approach. J Allergy Clin Immunol Pract 9:1423–1437. https://doi.org/10.1016/j.jaip.2020.12.047
Ulbricht J, Jordan R, Luxenhofer R (2014) On the biodegradability of polyethylene glycol, polypeptoids, and poly (2-oxazoline)s. Biomaterials 35:4848–4861. https://doi.org/10.1016/j.biomaterials.2014.02.029
Peckham H, de Gruijter NM, Raine C, Radziszewska A, Ciurtin C, Wedderburn LR, Rosser EC, Webb K, Deakin CT (2020) Male sex identified by global COVID-19 meta-analysis as a risk factor for death and ICU admission. Nat Commun 11:6317. https://doi.org/10.1038/s41467-020-19741-6
Saifer MG, Williams LD, Sobczyk MA, Michaels SJ, Sherman MR (2014) Selectivity of binding of PEGs and PEG-like oligomers to anti-PEG antibodies induced by methoxypolyethylene glycol-proteins. Mol Immunol 57:236–246. https://doi.org/10.1016/j.molimm.2013.07.014
Yang Q, Lai SK (2015) Anti-PEG immunity: emergence, characteristics, and unaddressed questions. Wiley Interdiscip Rev Nanomed Nanobiotechnol 7:655–677. https://doi.org/10.1002/wnan.1339
Zhang P, Sun F, Liu S, Jiang S (2016) Anti-PEG antibodies in the clinic: current issues and beyond PEGylation. J Control Release 244:184–193. https://doi.org/10.1016/j.jconrel.2016.06.040
Zhou Z-H, Stone CA, Jakubovic B, Phillips EJ, Sussman G, Park J, Hoang U, Kirshner SL, Levin R, Kozlowski S (2021) Anti-PEG IgE in anaphylaxis associated with polyethylene glycol. J Allergy Clin Immunol Pract 9:1731-1733.e3. https://doi.org/10.1016/j.jaip.2020.11.011
Stone CA Jr, Liu Y, Relling MV, Krantz MS, Pratt AL, Abreo A, Hemler JA, Phillips EJ (2019) Immediate hypersensitivity to polyethylene glycols and polysorbates: more common than we have recognized. J Allergy Clin Immunol Pract 7:1533-1540.e8. https://doi.org/10.1016/j.jaip.2018.12.003
Hershfield MS, Ganson NJ, Kelly SJ, Scarlett EL, Jaggers DA, Sundy JS (2014) Induced and pre-existing anti-polyethylene glycol antibody in a trial of every 3-week dosing of pegloticase for refractory gout, including in organ transplant recipients. Arthritis Res Ther 16:R63. https://doi.org/10.1186/ar4500
Market (2017) Pegloticase: withdrawal of its European marketing authorisation is welcome. Prescrire Int 26:71. https://www.ncbi.nlm.nih.gov/pubmed/30730621 , https://www.ema.europa.eu/en/documents/public-statement/public-statement-krystexxa-withdrawal-marketing-authorisation-european-union_en.pdf
Vrieze J (2020) Suspicions grow that nanoparticles in Pfizer’s COVID-19 vaccine trigger rare allergic reactions. Scienceinsider. https://doi.org/10.1126/science.abg2359 , https://www.science.org/content/article/suspicions-grow-nanoparticles-pfizer-s-covid-19-vaccine-trigger-rare-allergic-reactions . Accessed 21 Dec 2020
Coors EA, Seybold H, Merk HF, Mahler V (2005) Polysorbate 80 in medical products and non-immunologic anaphylactoid reactions. Ann Allergy Asthma Immunol 95:593–599. https://doi.org/10.1016/S1081-1206(10)61024-1
Carpenter T, Konig J, Hochfelder J, Siegel S, Gans M (2022) Polyethylene glycol and polysorbate testing in 12 patients before or after coronavirus disease 2019 vaccine administration. Ann Allergy Asthma Immunol 128:99–101. https://doi.org/10.1016/j.anai.2021.10.009
Sadoff J, Gray G, Vandebosch A, Cárdenas V, Shukarev G, Grinsztejn B, Goepfert PA, Truyers C, Fennema H, Spiessens B (2021) Safety and efficacy of single-dose Ad26.COV2.S vaccine against COVID-19. N Engl J Med 384:2187–2201. https://doi.org/10.1056/NEJMoa2101544
Baden LR, El Sahly HM, Essink B, Kotloff K, Frey S, Novak R, Diemert D, Spector SA, Rouphael N, Creech CB (2021) Efficacy and safety of the mRNA-1273 SARS-CoV-2 vaccine. N Engl J Med 384:403–416. https://doi.org/10.1056/NEJMoa2035389
Polack FP, Thomas SJ, Kitchin N, Absalon J, Gurtman A, Lockhart S, Perez JL, Marc GP, Moreira ED, Zerbini C (2020) Safety and efficacy of the BNT162b2 mRNA COVID-19 vaccine. N Engl J Med. https://doi.org/10.1056/NEJMoa2034577
Schwede K, Simon JC, Treudler R (2019) A case of severe immediate type reaction to macrogol and polysorbate 60 after intravaginal drug application. Eur J Dermatol 29:329–331. https://doi.org/10.1684/ejd.2019.3541
Wenande E, Garvey L (2016) Immediate-type hypersensitivity to polyethylene glycols: a review. Clin Exp Allergy 46:907–922. https://doi.org/10.1111/cea.12760
Rajpurkar M, O’Brien SH, Haamid FW, Cooper DL, Gunawardena S, Chitlur M (2016) Heavy menstrual bleeding as a common presenting symptom of rare platelet disorders: illustrative case examples. J Pediatr Adolesc Gynecol 29:537–541. https://doi.org/10.1016/j.jpag.2016.02.002
Soltani Hekmat A, Javanmardi K (2021) Possible risk of thrombotic events following Oxford-AstraZeneca COVID-19 vaccination in women receiving estrogen. BioMed Res Int 2021:7702863. https://doi.org/10.1155/2021/7702863
Hunter PR (2021) Thrombosis after COVID-19 vaccination. BMJ 373:n958. https://doi.org/10.1136/bmj.n958
Alghamdi AN, Alotaibi MI, Alqahtani AS, Al Aboud D, Abdel-Moneim AS (2021) BNT162b2 and ChAdOx1 SARS-CoV-2 post-vaccination side-effects among Saudi vaccinees. Front Med 8:1796. https://doi.org/10.3389/fmed.2021.760047
GOV.UK (2022) Coronavirus vaccine - summary of yellow card reporting in vigilance, safety alerts and guidance. https://www.gov.uk/government/publications/coronavirus-covid-19-vaccine-adverse-reactions/coronavirus-vaccine-summary-of-yellow-card-reporting . Updated 8 March 2023
Rela M, Jothimani D, Vij M, Rajakumar A, Rammohan A (2021) Auto-immune hepatitis following COVID vaccination. J Autoimmun 123:102688. https://doi.org/10.1016/j.jaut.2021.102688
Patrizio A, Ferrari SM, Antonelli A, Fallahi P (2021) A case of Graves’ disease and type 1 diabetes mellitus following SARS-CoV-2 vaccination. J Autoimmun 125:102738. https://doi.org/10.1016/j.jaut.2021.102738
Zavala-Miranda MF, González-Ibarra SG, Pérez-Arias AA, Uribe-Uribe NO, Mejia-Vilet JM (2021) New-onset systemic lupus erythematosus beginning as class V lupus nephritis after COVID-19 vaccination. Kidney Int 100:1340–1341. https://doi.org/10.1016/j.kint.2021.09.009
Waheed S, Bayas A, Hindi F, Rizvi Z, Espinosa PS (2021) Neurological complications of COVID-19: Guillain-Barre syndrome following Pfizer COVID-19 vaccine. Cureus 13:e13426. https://doi.org/10.7759/cureus.13426
Abramson M, Yu SM-W, Campbell KN, Chung M, Salem F (2021) IgA nephropathy after SARS-CoV-2 vaccination. Kidney Med 3:860–863. https://doi.org/10.1016/j.xkme.2021.05.002
Jara LJ, Vera-Lastra O, Mahroum N, Pineda C, Shoenfeld Y (2022) Autoimmune post-COVID vaccine syndromes: does the spectrum of autoimmune/inflammatory syndrome expand? Clin Rheumatol 41:1603–1609. https://doi.org/10.1007/s10067-022-06149-4
Aldén M, Olofsson Falla F, Yang D, Barghouth M, Luan C, Rasmussen M, De Marinis Y (2022) Intracellular reverse transcription of Pfizer-BioNTech COVID-19 mRNA vaccine BNT162b2 in vitro in human liver cell line. Curr Issues Mol Biol 44:1115–1126. https://doi.org/10.3390/cimb44030073
Merchant HA (2022) Comment on Aldén et al. Intracellular reverse transcription of Pfizer-BioNTech COVID-19 mRNA vaccine BNT162b2 in vitro in human liver cell line. Curr Issues Mol Biol 44:1661–1663. https://doi.org/10.3390/cimb44040113
Rijkers GT, van Overveld FJ (2021) The “original antigenic sin” and its relevance for SARS-CoV-2 (COVID-19) vaccination. Clin Immunol Commun 1:13–16. https://doi.org/10.1016/j.clicom.2021.10.001
Hoepel W, Chen H-J, Geyer CE, Allahverdiyeva S, Manz XD, de Taeye SW, Aman J, Mes L, Steenhuis M, Griffith GR (2021) High titers and low fucosylation of early human anti-SARS-CoV-2 IgG promote inflammation by alveolar macrophages. Sci Transl Med 13:eabf8654. https://doi.org/10.1126/scitranslmed.abf8654
Kurdoğlu Z (2021) Do the COVID-19 vaccines cause menstrual irregularities? Int J Womens Health Reprod Sci 9:158–159. https://doi.org/10.15296/ijwhr.2021.29
Bozkurt B, Kamat I, Hotez PJ (2021) Myocarditis with COVID-19 mRNA vaccines. Circulation 144:471–484. https://doi.org/10.1161/CIRCULATIONAHA.121.056135
Seephetdee C, Bhukhai K, Buasri N, Leelukkanaveera P, Lerdwattanasombat P, Manopwisedjaroen S, Phueakphud N, Kuhaudomlarp S, Saphire EO, Thitithanyanont A (2022) Broad neutralization of SARS-CoV-2 variants by circular mRNA producing vFlip-X spike in mice. https://doi.org/10.1101/2022.03.17.484759
ClinicalTrials.gov (2021) SARS-CoV-2-Spike-Ferritin-Nanoparticle (SPFN) vaccine with ALFQ adjuvant for prevention of COVID-19 in healthy adults. (NCT04784767). https://clinicaltrials.gov/ct2/show/NCT04784767
Joh DY, Zimmers Z, Avlani M, Heggestad JT, Aydin HB, Ganson N, Kumar S, Fontes CM, Achar RK, Hershfield MS (2019) Architectural modification of conformal PEG-bottlebrush coatings minimizes anti-PEG antigenicity while preserving stealth properties. Adv Healthc Mater 8:1801177. https://doi.org/10.1002/adhm.201801177
Friedl JD, Nele V, De Rosa G, Bernkop-Schnürch A (2021) Bioinert, stealth, or interactive: how surface chemistry of nanocarriers determines their fate in vivo. Adv Funct Mater 31:2103347. https://doi.org/10.1002/adfm.202103347
Han X, Lu Y, Xie J, Zhang E, Zhu H, Du H, Wang K, Song B, Yang C, Shi Y (2020) Zwitterionic micelles efficiently deliver oral insulin without opening tight junctions. Nat Nanotechnol 15:605–614. https://doi.org/10.1038/s41565-020-0693-6
Xue W, Liu Y, Zhang N, Yao Y, Ma P, Wen H, Huang S, Luo Y, Fan H (2018) Effects of core size and peg coating layer of iron oxide nanoparticles on the distribution and metabolism in mice. Int J Nanomedicine 13:5719–5728. https://doi.org/10.2147/IJN.S165451
Yao X, Qi C, Sun C, Huo F, Jiang X (2023) Poly(ethylene glycol) alternatives in biomedical applications. Nano Today 48:101738. https://doi.org/10.1016/j.nantod.2022.101738
Patente TA, Pinho MP, Oliveira AA, Evangelista GC, Bergami-Santos PC, Barbuto JA (2019) Human dendritic cells: their heterogeneity and clinical application potential in cancer immunotherapy. Front Immunol 9:3176. https://doi.org/10.3389/fimmu.2018.03176
Tezera LB, Bielecka MK, Chancellor A, Reichmann MT, Shammari BA, Brace P, Batty A, Tocheva A, Jogai S, Marshall BG (2017) Dissection of the host-pathogen interaction in human tuberculosis using a bioengineered 3-dimensional model. Elife 6:e21283. https://doi.org/10.7554/eLife.21283
Geisbert TW, Pushko P, Anderson K, Smith J, Davis KJ, Jahrling PB (2002) Evaluation in nonhuman primates of vaccines against Ebola virus. Emerg Infect Dis 8:503–507. https://doi.org/10.3201/eid0805.010284
Vidal Oribe I, Venturini Díaz M, Hernández Alfonso P, del Pozo Gil M, González Mahave I, Lobera Labairu T (2022) Tolerance of SARS-CoV-2 vaccines with polyethylene glycol in allergic patients to polysorbate 80. J Investig Allergol Clin Immunol 32:403–405. https://doi.org/10.18176/jiaci.0772
Broyles AD, Banerji A, Barmettler S, Biggs CM, Blumenthal K, Brennan PJ, Breslow RG, Brockow K, Buchheit KM, Cahill KN (2020) Practical guidance for the evaluation and management of drug hypersensitivity: specific drugs. J Allergy Clin Immunol Pract 8:S16–S116. https://doi.org/10.1016/j.jaip.2020.08.002
Greenhawt M, Shaker M, Golden DB (2021) Peg/polysorbate skin testing has no utility in the assessment of suspected allergic reactions to SARS-CoV-2 vaccines. J Allergy Clin Immunol Pract 9:3321–3322. https://doi.org/10.1016/j.jaip.2021.06.025
Pitlick MM, Gonzalez-Estrada A, Park MA (2022) Graded COVID-19 vaccine administration: a safe alternative to vaccine avoidance. Ann Allergy Asthma Immunol 128:731–733. https://doi.org/10.1016/j.anai.2022.02.024
Liu X, Shaw RH, Stuart AS, Greenland M, Aley PK, Andrews NJ, Cameron JC, Charlton S, Clutterbuck EA, Collins AM (2021) Safety and immunogenicity of heterologous versus homologous prime-boost schedules with an adenoviral-vectored and mRNA COVID-19 vaccine (Com-COV): a single-blind, randomized, non-inferiority trial. Lancet 398:856–869. https://doi.org/10.1016/S0140-6736(21)01694-9
Yii A, Tay TR, Choo X, Koh M, Tee A, Wang DY (2018) Precision medicine in united airways disease: a “treatable traits” approach. Allergy 73:1964–1978. https://doi.org/10.1111/all.13496
Łoczechin A, Séron K, Barras A, Giovanelli E, Belouzard S, Chen Y-T, Metzler-Nolte N, Boukherroub R, Dubuisson J, Szunerits S (2019) Functional carbon quantum dots as medical countermeasures to human coronavirus. ACS Appl Mater Interfaces 11:42964–42974. https://doi.org/10.1021/acsami.9b15032
Huang X, Li M, Xu Y, Zhang J, Meng X, An X, Sun L, Guo L, Shan X, Ge J (2019) Novel gold nanorod-based HR1 peptide inhibitor for Middle East respiratory syndrome coronavirus. ACS Appl Mater Interfaces 11:19799–19807. https://doi.org/10.1021/acsami.9b04240
Neufurth M, Wang X, Tolba E, Lieberwirth I, Wang S, Schröder HC, Müller WE (2020) The inorganic polymer, polyphosphate, blocks binding of SARS-CoV-2 spike protein to ACE2 receptor at physiological concentrations. Biochem Pharmacol 182:114215. https://doi.org/10.1016/j.bcp.2020.114215
Tang H, Qin H, He S, Li Q, Xu H, Sun M, Li J, Lu S, Luo S, Mao P (2023) Anti-coronaviral nanocluster restrain infections of SARS-CoV-2 and associated mutants through virucidal inhibition and 3CL protease inactivation. Adv Sci 10:2207098. https://doi.org/10.1002/advs.202207098
Gattani V, Dawre S (2023) Development of favipiravir loaded PLGA nanoparticles entrapped in in-situ gel for treatment of COVID-19 via nasal route. J Drug Deliv Sci Technol 79:104082. https://doi.org/10.1016/j.jddst.2022.104082
Idris A, Davis A, Supramaniam A, Acharya D, Kelly G, Tayyar Y, West N, Zhang P, McMillan CL, Soemardy C (2021) A SARS-CoV-2 targeted siRNA-nanoparticle therapy for COVID-19. Mol Ther 29:2219–2226. https://doi.org/10.1016/j.ymthe.2021.05.004
Nadworny PL, Hickerson L, Holley-Harrison HD, Bloom DC, Grams TR, Edwards TG, Schultz GS, Burrell RE (2023) Treatment of infection and inflammation associated with COVID-19, multi-drug resistant pneumonia and fungal sinusitis by nebulizing a nanosilver solution. Nanomed Nanotechnol Biol Med 48:102654. https://doi.org/10.1016/j.nano.2023.102654
Saify Nabiabad H, Amini M, Demirdas S (2022) Specific delivering of RNAi using spike’s aptamer-functionalized lipid nanoparticles for targeting SARS-CoV-2: a strong anti-COVID drug in a clinical case study. Chem Biol Drug Des 99:233–246. https://doi.org/10.1111/cbdd.13978
Pokhrel LR, Williams F, Cook PP, O’Rourke D, Murray G, Akula SM (2022) Preclinical efficacy and safety of novel SNAT against SARS-CoV-2 using a hamster model. Drug Delivery Transl Res 12:3007–3016. https://doi.org/10.1007/s13346-022-01166-x
Zhou Y, Jiang X, Tong T, Fang L, Wu Y, Liang J, Xiao S (2020) High antiviral activity of mercaptoethane sulfonate functionalized Te/BSA nanostars against arterivirus and coronavirus. RSC Adv 10:14161–14169. https://doi.org/10.1039/D0RA01387K
Du T, Zhang J, Li C, Song T, Li P, Liu J, Du X, Wang S (2020) Gold/silver hybrid nanoparticles with enduring inhibition of coronavirus multiplication through multisite mechanisms. Bioconjug Chem 31:2553–2563. https://doi.org/10.1021/acs.bioconjchem.0c00506
Du T, Liang J, Dong N, Lu J, Fu Y, Fang L, Xiao S, Han H (2018) Glutathione-capped Ag2S nanoclusters inhibit coronavirus proliferation through blockage of viral RNA synthesis and budding. ACS Appl Mater Interfaces 10:4369–4378. https://doi.org/10.1021/acsami.7b13811
Hu C-MJ, Chang W-S, Fang Z-S, Chen Y-T, Wang W-L, Tsai H-H, Chueh L-L, Takano T, Hohdatsu T, Chen H-W (2017) Nanoparticulate vacuolar ATPase blocker exhibits potent host-targeted antiviral activity against feline coronavirus. Sci Rep 7:1343. https://doi.org/10.1038/s41598-017-13316-0
Jensen DMK, Cun D, Maltesen MJ, Frøkjaer S, Nielsen HM, Foged C (2010) Spray drying of siRNA-containing PLGA nanoparticles intended for inhalation. J Control Release 142:138–145. https://doi.org/10.1016/j.jconrel.2009.10.010
Lv X, Wang P, Bai R, Cong Y, Suo S, Ren X, Chen C (2014) Inhibitory effect of silver nanomaterials on transmissible virus-induced host cell infections. Biomaterials 35:4195–4203. https://doi.org/10.1016/j.biomaterials.2014.01.054
Valizadeh H, Abdolmohammadi-Vahid S, Danshina S, Gencer MZ, Ammari A, Sadeghi A, Roshangar L, Aslani S, Esmaeilzadeh A, Ghaebi M (2020) Nano-curcumin therapy, a promising method in modulating inflammatory cytokines in COVID-19 patients. Int Immunopharmacol 89:107088. https://doi.org/10.1016/j.intimp.2020.107088
Park HH, Park W, Lee YY, Kim H, Seo HS, Choi DW, Kwon HK, Na DH, Kim TH, Choy YB (2020) Bioinspired DNase-I-coated melanin-like nanospheres for modulation of infection-associated NETosis dysregulation. Adv Sci 7:2001940. https://doi.org/10.1002/advs.202001940
Lee YY, Park HH, Park W, Kim H, Jang JG, Hong KS, Lee J-Y, Seo HS, Na DH, Kim TH, Choy YB (2021) Long-acting nanoparticulate DNase-1 for effective suppression of SARS-CoV-2-mediated neutrophil activities and cytokine storm. Biomaterials 267:120389. https://doi.org/10.1016/j.biomaterials.2020.120389
Morad R, Akbari M, Rezaee P, Koochaki A, Maaza M, Jamshidi Z (2021) First principle simulation of coated hydroxychloroquine on Ag, Au, and Pt nanoparticles. Sci Rep 11:1–9. https://doi.org/10.1038/s41598-021-81617-6
Niemiec S, Hilton S, Wallbank A, Azeltine-Bannerman M, Louiselle A, Elajaili H, Allawzi A, Xu J, Mattson C, Dewberry L (2020) Cerium oxide nanoparticles conjugated to anti-inflammatory microRNA-146a prevent bleomycin-induced acute lung injury. Nanomed Nanotechnol Biol Med 34:102388. https://doi.org/10.1016/j.nano.2021.102388
Wang G, Gaikwad H, McCarthy M, Gonzalez-Juarrero M, Li Y, Armstrong M, Reisdorph N, Morrison T, Simberg D (2021) Lipid nanoparticle formulation of niclosamide (Nano NCM) effectively inhibits SARS-CoV-2 replication in vitro. Precis Nanomed 4:724–737. https://doi.org/10.33218/001c.18813
Zhang Q, Honko A, Zhou J, Gong H, Downs SN, Vasquez JH, Fang RH, Gao W, Griffiths A, Zhang L (2020) Cellular nanosponges inhibit SARS-CoV-2 infectivity. Nano Lett 20:5570–5574. https://doi.org/10.1021/acs.nanolett.0c02278
Starpharma (2020) SPL creates slow-release soluble DEP® remdesivir nanoparticle. https://starpharma.com/assets/asxannouncements/200901%20SPL%20creates%20slow%20release%20soluble%20DEP%20remdesivir%20nanoparticle.pdf
Khaitov M, Nikonova A, Shilovskiy I, Kozhikhova K, Kofiadi I, Vishnyakova L, Nikolskii A, Gattinger P, Kovchina V, Barvinskaia E (2021) Silencing of SARS-CoV-2 with modified siRNA-peptide dendrimer formulation. Allergy 76:2840–2854. https://doi.org/10.1111/all.14850
ClinicalTrials.gov (2021) Treatment of patients with mild coronavirus-19 (COVID-19) disease with methotrexate associated to LDL like nanoparticles (Nano-COVID19). (NCT04610567). https://clinicaltrials.gov/ct2/show/NCT04610567?term=nanoparticle&recrs=adeh&cond=COVID-19&draw=2&rank=5
ClinicalTrials.gov (2020) Efficacy and safety of MTX-loaded nanoparticles to treat severe COVID-19 patients. (NCT04352465). https://clinicaltrials.gov/ct2/show/study/NCT04352465?term=nanoparticle&recrs=abdeh&cond=COVID-19&draw=2&rank=1
ClinicalTrials.gov (2021) Colloidal silver, treatment of COVID-19. (NCT04978025). https://clinicaltrials.gov/ct2/show/NCT04978025?term=nanoparticle&recrs=abdeh&cond=COVID-19&draw=3&rank=19
Gambichler T, Hamdani N, Budde H, Sieme M, Skrygan M, Scholl L, Dickel H, Behle B, Ganjuur N, Scheel C (2021) Bullous pemphigoid after SARS-CoV-2 vaccination: spike protein-directed immunofluorescence confocal microscopy and T cell receptor studies. Br J Dermatol 186:1012–1013. https://doi.org/10.1111/bjd.20890
Cemşitoğlu N, Adışen E, Erdem Ö (2022) Lichen planus after CoronaVac: a rare complication of vaccines. J Eur Acad Dermatol Venereol 36:e202–e204. https://doi.org/10.1111/jdv.17906
Alghamdi FA, Khayyat ST, Alshareef MM, Wa F (2022) New-onset lichen planusiInduced by the Pfizer COVID-19 vaccine. Case reports in dermatological medicine New-onset cutaneous lichen planus triggered by COVID-19 vaccination. Case Rep Dermatol Med 2022:2082445. https://doi.org/10.1155/2022/2082445
Troeltzsch M, Berndt R (2021) Comment on “oral lichen planus following the administration of vector-based COVID-19 vaccine (Ad26. Cov2. S).” Authors’ reply Oral Dis 27:1327–1328. https://doi.org/10.1111/odi.14060
Kha C, Itkin A (2021) New-onset chilblains in close temporal association with mRNA-1273 (Moderna) vaccination. JAAD Case Reports 8:66–67. https://doi.org/10.1016/j.jdcr.2021.03.046
Toom S, Wolf B, Avula A, Peeke S, Becker K (2021) Familial thrombocytopenia flare-up following the first dose of mRNA-1273 COVID-19 vaccine. Am J Hematol 96:E107–E108. https://doi.org/10.1002/Fajh.26128
McMahon DE, Kovarik CL, Damsky W, Rosenbach M, Lipoff JB, Tyagi A, Chamberlin G, Fathy R, Nazarian RM, Desai SR (2022) Clinical and pathologic correlation of cutaneous COVID-19 vaccine reactions including V-REPP: a registry-based study. J Am Acad Dermatol 86:113–121. https://doi.org/10.1016/j.jaad.2021.09.002
Ackerman M, Henry D, Finon A, Binois R, Esteve E (2021) Persistent maculopapular rash after the first dose of Pfizer-BioNTech COVID-19 vaccine. J Eur Acad Dermatol Venereol 35:e430–e432. https://doi.org/10.1111/jdv.17248
Cyrenne B, Al-Mohammedi F, DeKoven J, Alhusayen R (2021) Pityriasis rosea-like eruptions following vaccination with BNT162b2 mRNA COVID-19 vaccine. J Eur Acad Dermatol Venereol 35:e485–e487. https://doi.org/10.1111/jdv.17342
Balingit A (2023) What is COVID arm? Healthline.com. https://www.healthline.com/health/adult-vaccines/covid-arm
Cirillo N (2021) Reported orofacial adverse effects of COVID-19 vaccines: the knowns and the unknowns. J Oral Pathol Med 50:424–427. https://doi.org/10.1111/jop.13165
Furer V, Zisman D, Kibari A, Rimar D, Paran Y, Elkayam O (2021) Herpes zoster following BNT162b2 mRNA COVID-19 vaccination in patients with autoimmune inflammatory rheumatic diseases: a case series. Rheumatology (Oxford) 60:e8–e9. https://doi.org/10.1093/rheumatology/keab345
Patlolla AK, Hackett D, Tchounwou PB (2015) Genotoxicity study of silver nanoparticles in bone marrow cells of Sprague-Dawley rats. Food Chem Toxicol 85:52–60. https://doi.org/10.1016/j.fct.2015.05.005
Yin N, Liu Q, Liu J, He B, Cui L, Li Z, Yun Z, Qu G, Liu S, Zhou Q (2013) Silver nanoparticle exposure attenuates the viability of rat cerebellum granule cells through apoptosis coupled to oxidative stress. Small 9:1831–1841. https://doi.org/10.1002/smll.201202732
Ziemińska E, Stafiej A, Strużyńska L (2014) The role of the glutamatergic NMDA receptor in nanosilver-evoked neurotoxicity in primary cultures of cerebellar granule cells. Toxicology 315:38–48. https://doi.org/10.1016/j.tox.2013.11.008
Sung JH, Ji JH, Park JD, Yoon JU, Kim DS, Jeon KS, Song MY, Jeong J, Han BS, Han JH (2009) Subchronic inhalation toxicity of silver nanoparticles. Toxicol Sci 108:452–461. https://doi.org/10.1186/1743-8977-7-20
Jia J, Li F, Zhou H, Bai Y, Liu S, Jiang Y, Jiang G, Yan B (2017) Oral exposure to silver nanoparticles or silver ions may aggravate fatty liver disease in overweight mice. Environ Sci Technol 51:9334–9343. https://doi.org/10.1021/acs.est.7b02752
Kim YS, Kim JS, Cho HS, Rha DS, Kim JM, Park JD, Choi BS, Lim R, Chang HK, Chung YH (2008) Twenty-eight-day oral toxicity, genotoxicity, and gender-related tissue distribution of silver nanoparticles in Sprague-Dawley rats. Inhal Toxicol 20:575–583. https://doi.org/10.1080/08958370701874663
Van der Zande M, Vandebriel RJ, Van Doren E, Kramer E, Herrera Rivera Z, Serrano-Rojero CS, Gremmer ER, Mast J, Peters RJ, Hollman PC (2012) Distribution, elimination, and toxicity of silver nanoparticles and silver ions in rats after 28-day oral exposure. ACS Nano 6:7427–7442. https://doi.org/10.1021/nn302649p
Trickler WJ, Lantz SM, Murdock RC, Schrand AM, Robinson BL, Newport GD, Schlager JJ, Oldenburg SJ, Paule MG, Slikker W Jr (2010) Silver nanoparticle-induced blood-brain barrier inflammation and increased permeability in primary rat brain microvessel endothelial cells. Toxicol Sci 118:160–170. https://doi.org/10.1093/toxsci/kfq244
Juling S, Böhmert L, Lichtenstein D, Oberemm A, Creutzenberg O, Thünemann AF, Braeuning A, Lampen A (2018) Comparative proteomic analysis of hepatic effects induced by nanosilver, silver ions, and nanoparticle coating in rats. Food Chem Toxicol 113:255–266. https://doi.org/10.1016/j.fct.2018.01.056
Zhurkov V, Savostikova O, Yurchenko V, Krivtsova E, Kovalenko M, Murav’eva L, Alekseeva A, Belyaeva N, Mikhailova R, Sycheva L (2017) Features of the mutagenic and cytotoxic effects of nanosilver and silver sulfate in mice. Nanotechnol 12:667–672. https://doi.org/10.1134/S1995078017060143
Pratsinis A, Hervella P, Leroux JC, Pratsinis SE, Sotiriou GA (2013) Toxicity of silver nanoparticles in macrophages. Small 9:2576–2584. https://doi.org/10.1002/smll.201202120
Pang S, Gao Y, Wang F, Wang Y, Cao M, Zhang W, Liang Y, Song M, Jiang G (2020) Toxicity of silver nanoparticles on wound healing: a case study of zebrafish fin regeneration model. Sci Total Environ 717:137178. https://doi.org/10.1016/j.scitotenv.2020.137178
Wen H, Dan M, Yang Y, Lyu J, Shao A, Cheng X, Chen L, Xu L (2017) Acute toxicity and genotoxicity of silver nanoparticle in rats. Plos One 12:e0185554. https://doi.org/10.1371/Fjournal.pone.0185554
Lu T, Qu Q, Lavoie M, Pan X, Peijnenburg W, Zhou Z, Pan X, Cai Z, Qian H (2020) Insights into the transcriptional responses of a microbial community to silver nanoparticles in a freshwater microcosm. Environ Pollut 258:113727. https://doi.org/10.1016/j.envpol.2019.113727
Sung JH, Ji JH, Yoon JU, Kim DS, Song Y, Jeong J, Han S, Han JH, Chung YH, Kim J (2008) Lung function changes in Sprague-Dawley rats after prolonged inhalation exposure to silver nanoparticles. Inhalation Toxicol 20:567–574. https://doi.org/10.1080/08958370701874671
Lee S-H, Hwang SH, Park JS, Park H-S, Shin YS (2016) Anaphylaxis to polyethylene glycol (Colyte®) in a patient with diverticulitis. J Korean Med Sci 31:1662–1663. https://doi.org/10.3346/jkms.2016.31.10.1662
Pidaparti M, Bostrom B (2012) Comparison of allergic reactions to Pegasparaginase given intravenously versus intramuscularly. Pediatr Pediatr Blood Cancer 59:436–439. https://doi.org/10.1002/pbc.23380
Moreno A, Pitoc GA, Ganson NJ, Layzer JM, Hershfield MS, Tarantal AF, Sullenger BA (2019) Anti-PEG antibodies inhibit the anticoagulant activity of pegylated aptamers. Cell Chem Biol 26:634-644.e3. https://doi.org/10.1016/j.chembiol.2019.02.001
Sung JH, Ji JH, Park JD, Song MY, Song KS, Ryu HR, Yoon JU, Jeon KS, Jeong J, Han BS (2011) Subchronic inhalation toxicity of gold nanoparticles. Part Fibre Toxicol 8:1–18. https://doi.org/10.1186/1743-8977-8-16
Kim K-T, Zaikova T, Hutchison JE, Tanguay RL (2013) Gold nanoparticles disrupt zebrafish eye development and pigmentation. Toxicol Sci 133:275–288. https://doi.org/10.1093/toxsci/kft081
Hext PM, Tomenson JA, Thompson P (2005) Titanium dioxide: Inhalation toxicology and epidemiology. Ann Occup Hyg 49:461–472. https://doi.org/10.1093/annhyg/mei012
Zhang L, Bai R, Li B, Ge C, Du J, Liu Y, Le Guyader L, Zhao Y, Wu Y, He S (2011) Rutile TiO 2 particles exert size and surface coating dependent retention and lesions on the murine brain. Toxicol Lett 207:73–81. https://doi.org/10.1016/j.toxlet.2011.08.001
Wang J, Li Y, Li W, Chen C, Li B, Zhao Y (2008) Biological effect of intranasally instilled titanium dioxide nanoparticles on female mice. NANO 3:279–285. https://doi.org/10.1142/S1793292008001325
Mohammadipour A, Fazel A, Haghir H, Motejaded F, Rafatpanah H, Zabihi H, Hosseini M, Bideskan AE (2014) Maternal exposure to titanium dioxide nanoparticles during pregnancy; impaired memory and decreased hippocampal cell proliferation in rat offspring. Environ Toxicol Pharmacol 37:617–625. https://doi.org/10.1016/j.etap.2014.01.014
Hong F, Ji L, Zhou Y, Wang L (2017) Retracted: pulmonary fibrosis of mice and its molecular mechanism following chronic inhaled exposure to TiO2 nanoparticles. Environ Toxicol 33:711. https://doi.org/10.1002/TOX.22493
Chen J, Dong X, Zhao J, Tang G (2009) In vivo acute toxicity of titanium dioxide nanoparticles to mice after intraperitoneal injection. J Appl Toxicol 29:330–337. https://doi.org/10.1002/jat.1414
Download references
Acknowledgements
The authors thank Dr. Jianfeng Jin and Prof. Jenneke Klein-Nulend for their outstanding help in editing the manuscript. Also, we thank David Alexander for his careful reading of the manuscript.
Author information
Authors and affiliations.
Department of Medical Biotechnology, School of Medicine, Shahid Sadoughi University of Medical Sciences, Yazd, Iran
Fatemeh Araste
Department of Oral Cell Biology, Academic Centre for Dentistry Amsterdam (ACTA), University of Amsterdam and Vrije Universiteit Amsterdam, Amsterdam Movement Sciences, Gustav Mahlerlaan, 3004, 1081 LA, Amsterdam, The Netherlands
Astrid Diana Bakker & Behrouz Zandieh-Doulabi
You can also search for this author in PubMed Google Scholar
Contributions
B. Zandieh-Doulabi had the idea for the article. F. Araste performed the literature search and data analysis. F. Araste and B. Zandieh-Doulabi drafted the work, and all authors critically revised it.
Corresponding author
Correspondence to Behrouz Zandieh-Doulabi .
Ethics declarations
Conflict of interest.
The authors declare no competing interests.
Additional information
Publisher's note.
Springer Nature remains neutral with regard to jurisdictional claims in published maps and institutional affiliations.
Rights and permissions
Open Access This article is licensed under a Creative Commons Attribution 4.0 International License, which permits use, sharing, adaptation, distribution and reproduction in any medium or format, as long as you give appropriate credit to the original author(s) and the source, provide a link to the Creative Commons licence, and indicate if changes were made. The images or other third party material in this article are included in the article's Creative Commons licence, unless indicated otherwise in a credit line to the material. If material is not included in the article's Creative Commons licence and your intended use is not permitted by statutory regulation or exceeds the permitted use, you will need to obtain permission directly from the copyright holder. To view a copy of this licence, visit http://creativecommons.org/licenses/by/4.0/ .
Reprints and permissions
About this article
Araste, F., Bakker, A.D. & Zandieh-Doulabi, B. Potential and risks of nanotechnology applications in COVID-19-related strategies for pandemic control. J Nanopart Res 25 , 229 (2023). https://doi.org/10.1007/s11051-023-05867-3
Download citation
Received : 09 December 2022
Accepted : 09 October 2023
Published : 09 November 2023
DOI : https://doi.org/10.1007/s11051-023-05867-3
Share this article
Anyone you share the following link with will be able to read this content:
Sorry, a shareable link is not currently available for this article.
Provided by the Springer Nature SharedIt content-sharing initiative
- Antiviral strategies
- Health effects
- Nanoparticles
- Nanoparticle-associated risks
- Nanotechnology
- Pandemic control
- Find a journal
- Publish with us
- Track your research
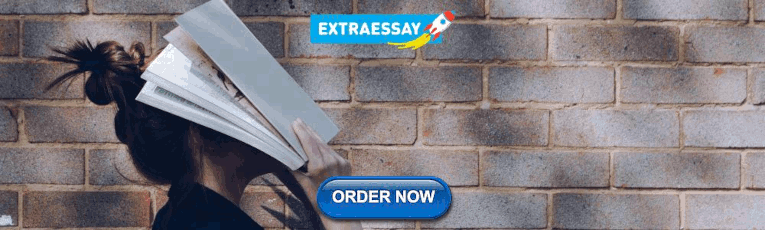
IMAGES
VIDEO
COMMENTS
A peer-reviewed journal that covers synthesis, assembly, transport, reactivity, and stability of nanoscale structures. Features applications, structures, and devices with novel functions via precursor nanoparticles. See latest articles, impact factor, submission to first decision, and more.
The Journal of Nanoparticle Research is a monthly peer-reviewed scientific journal published by Springer Science+Business Media. It focuses mainly on physical, chemical, and biological phenomena and processes in structures of sizes comparable to a few nanometers.
Find out the impact, ranking, and scope of the Journal of Nanoparticle Research, a peer-reviewed journal covering the physical, chemical and biological phenomena and processes of nanoparticles and nanostructures. See the quartiles, categories, and publications of the journal based on the SJR score and the year of publication.
Journal of Nanoparticle Research. Published by Springer Nature. Online ISSN: 1572-896X ...
The Journal of Nanoparticle Research is a monthly peer-reviewed journal that explores the specific concepts, properties, phenomena and processes of structures at the nanoscale. Coverage includes synthesis, assembly, transport, reactivity, and stability, and emphasizes realization and application of systems, structures and devices with novel ...
ScienceGate provides access to the latest publications, citations, and keywords of Journal of Nanoparticle Research, a peer-reviewed journal by Springer-Verlag. Browse the articles on topics such as polymeric nanoparticles, drug delivery, cobalt nanoparticles, and more.
Journal of Nanoparticle Research. Published by Springer Nature. Online ISSN: 1572-896X. Print ISSN: 1388-0764. Learn more about this page. Share on Twitter. Recent publications +3.
The objective of the Journal of Nanoparticle Research is to disseminate knowledge of the physical, chemical and biological phenomena and processes in structures that have at least one lengthscale ranging from molecular to approximately 100 nm (or submicron in some situations), and exhibit improved and novel properties that are a direct result of their small size.
The principle motivation to perform research on nanoparticles is founded in the so-called quantum size effect. Metal and semiconducting nanoparticles just a few nanometers in diameter, and thus with sizes somewhere between single atoms/molecules and bulk materials, show pronounced size- (and also shape-) dependent electronic and optical properties [12], [13], [14].
2.2. Discovery of C, Ag, Zn, Cu, and Au nanoparticles. Carbon NPs were found in 1991, and Iijima and Ichihashi announced the single-wall carbon nanotube synthesis with a diameter of 1 nanometer in 1993 (Chen et al., 2021).Carbon nanotubes (CNTs), also known as Bucky tubes, are a kind of nanomaterial made up of a two-dimensional hexagonal lattice of carbon atoms.
Nanotechnology is a known field of research since last century. Since "nanotechnology" was presented by Nobel laureate Richard P. Feynman during his well famous 1959 lecture "There's Plenty of Room at the Bottom" (Feynman, 1960), there have been made various revolutionary developments in the field of nanotechnology.Nanotechnology produced materials of various types at nanoscale level.
Nanoparticles are particles that exist on a nanometre scale (i.e., below 100 nm in at least one dimension). They can possess physical properties such as uniformity, conductance or special optical ...
Journal of Nanoparticle Research has an h-index of 137.It means 137 articles of this journal have more than 137 number of citations. The h-index is a way of measuring the productivity and citation impact of the publications. The h-index is defined as the maximum value of h such that the given journal/author has published h papers that have each been cited at least h number of times.
Journal of nanoparticle research (Dordrecht. Online) Identifiers. ISSN : 1572-896X. Linking ISSN (ISSN-L): 1388-0764. Resource information Archival Status. Title proper: Journal of nanoparticle research. Country: Netherlands. Medium: Online. Status Publisher Keeper From To Updated Extent of archive; Preserved. Springer. CLOCKSS Archive. 1999.
Inderscience is a global company, a dynamic leading independent journal publisher disseminates the latest research across the broad fields of science, engineering and technology; management, public and business administration; environment, ecological economics and sustainable development; computing, ICT and internet/web services, and related areas.
Find out the quartile, impact factor, citation and open access status of JOURNAL OF NANOPARTICLE RESEARCH, a materials science, chemistry and nanoscience journal published by Springer-Verlag. The journal is ranked Q3 in WoS core and Q4 in WoS quartile.
Journal of Nanoparticle Research is a peer-reviewed journal that delves into concepts, properties, phenomena, and processes of structures at the nanoscale. Covered topics include synthesis, assembly, transport, reactivity, and stability of nanoscale structures. Features applications, structures, and devices with novel functions via precursor ...
International Scientific Journal & Country Ranking. SCImago Institutions Rankings SCImago Media Rankings SCImago Iber SCImago Research Centers Ranking SCImago Graphica Ediciones Profesionales de la Información
Journal Article Facet-Dependent Dispersion and Aggregation of Aqueous Hematite Nanoparticles Share: Share on Facebook Share on X (formerly Twitter) Share on LinkedIn Email To:
The present work was supported by research grants from the Social Developmental Project of Jiangsu Province (BE2023848), the National Natural Science Foundation of China (41907135), and the Hydraulic Science and Technology Project of Jiangsu Province (2021078).
Throughout the twentieth century, viral infections significantly impacted global health, causing millions of deaths worldwide. To combat these diseases, nanotechnology has emerged as a promising approach in the development of antiviral agents such as biosensors, nanoprobes, virus-like particles (VLPs), and functionalized nanoparticles [1,2,3].The COVID-19 pandemic highlighted the importance of ...