
An official website of the United States government
The .gov means it’s official. Federal government websites often end in .gov or .mil. Before sharing sensitive information, make sure you’re on a federal government site.
The site is secure. The https:// ensures that you are connecting to the official website and that any information you provide is encrypted and transmitted securely.
- Publications
- Account settings
Preview improvements coming to the PMC website in October 2024. Learn More or Try it out now .
- Advanced Search
- Journal List

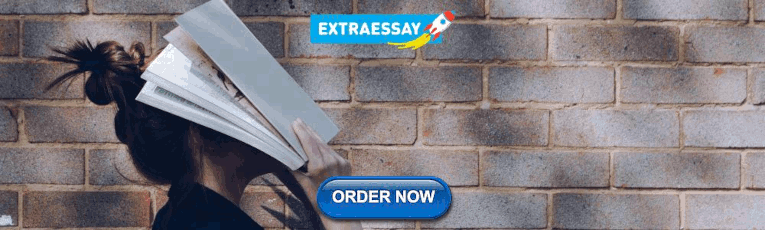
A Comprehensive Review for Groundwater Contamination and Remediation: Occurrence, Migration and Adsorption Modelling
Osamah al-hashimi.
1 Babylon Water Directorate, Babylon 51001, Iraq
2 School of Civil Engineering and Built Environment, Liverpool John Moores University, Liverpool L3 3AF, UK; [email protected] (K.H.); [email protected] (E.L.); [email protected] (T.M.Č.)
Khalid Hashim
3 Department of Environmental Engineering, College of Engineering, University of Babylon, Babylon 51001, Iraq
Edward Loffill
Tina marolt Čebašek, ismini nakouti.
4 Built Environment and Sustainable Technology Research Institute, Liverpool John Moores University, Byrom Street, Liverpool L3 3AF, UK; [email protected]
Ayad A. H. Faisal
5 Department of Environmental Engineering, College of Engineering, University of Baghdad, Baghdad 10001, Iraq; moc.oohay@lasiafazmahladebadaya
Nadhir Al-Ansari
6 Department of Civil, Environmental and Natural Resources Engineering, Lulea University of Technology, 97187 Lulea, Sweden; [email protected]
Associated Data
The data presented in this study are included in the article. Further inquiries can be directed to the corresponding author.
The provision of safe water for people is a human right; historically, a major number of people depend on groundwater as a source of water for their needs, such as agricultural, industrial or human activities. Water resources have recently been affected by organic and/or inorganic contaminants as a result of population growth and increased anthropogenic activity, soil leaching and pollution. Water resource remediation has become a serious environmental concern, since it has a direct impact on many aspects of people’s lives. For decades, the pump-and-treat method has been considered the predominant treatment process for the remediation of contaminated groundwater with organic and inorganic contaminants. On the other side, this technique missed sustainability and the new concept of using renewable energy. Permeable reactive barriers (PRBs) have been implemented as an alternative to conventional pump-and-treat systems for remediating polluted groundwater because of their effectiveness and ease of implementation. In this paper, a review of the importance of groundwater, contamination and biological, physical as well as chemical remediation techniques have been discussed. In this review, the principles of the permeable reactive barrier’s use as a remediation technique have been introduced along with commonly used reactive materials and the recent applications of the permeable reactive barrier in the remediation of different contaminants, such as heavy metals, chlorinated solvents and pesticides. This paper also discusses the characteristics of reactive media and contaminants’ uptake mechanisms. Finally, remediation isotherms, the breakthrough curves and kinetic sorption models are also being presented. It has been found that groundwater could be contaminated by different pollutants and must be remediated to fit human, agricultural and industrial needs. The PRB technique is an efficient treatment process that is an inexpensive alternative for the pump-and-treat procedure and represents a promising technique to treat groundwater pollution.
1. Introduction
Earth is known as the blue planet or the water planet because of the reality that most of its surface is covered by water, and it is the only planet in the solar system that has this huge quantity of water [ 1 , 2 ]. For various authorities and agencies dealing with water problems, the conservation of surface and groundwater purity without pollution is indeed an aim. In addition, groundwater is the main potable water supply used in many nations; this is also water for agriculture and industry [ 3 , 4 ]. The effect of global warming, climate change, the rise in weather temperature and evaporation increment, population growth, excessive use of fresh water in agriculture and industrial activities have all led to increasing reliance on groundwater [ 5 , 6 ]. Groundwater became fundamental for social and economic development. It is the sole source for drinking to about 2.5 billion people around the world [ 7 ]. There are many reasons to develop groundwater, but among the most important are [ 8 ]:
- (1) Groundwater usually lies in underground natural reservoirs. This promotes groundwater as a convenient source of water. Additionally, groundwater can be found in different quantities depending on aquifer capacity. Many times, aquifers detaining water larger than many human-made reservoirs; for example, the Ogalalla aquifer located in the United States produced up to 500 Km 3 of water for four decades, which is larger than Nasser lake in Egypt. The huge quantities of groundwater give an ability to pump water during the drought period, while surface water (in some places) is unable to be pumped in these quantities or at such high quality during such period.
- (2) In many cases, groundwater quality is better than surface water. This is due to the ability of aquifers to provide natural protection for groundwater from contamination.
- (3) Groundwater is a cheap, reliable source of water. It can be pumped out using small capital and can be drilled close to the location needed for water. Additionally, groundwater can be easily organized, managed and developed. For example, individuals can easily construct and operate their groundwater well on their land.
Pumping and treatment is a common technique used for groundwater treatment; however, the lack of groundwater quality restoration in the long term has been demonstrated in this method. An innovative approach to groundwater remediation is, therefore, necessary. The permeable reactive barrier (PRB) is proven as a promising technology for groundwater treatment by an interaction between the reactive material and the contaminant when the dissolved compounds migrate. In the permeable reactive barrier (PRB), water moves in a natural gradient, and no further energy is used to achieve the treatment [ 9 ]. The PRB is classified as in situ treatment, and the contaminant is transformed in the contaminated site into less toxic or immovable forms. The key benefits of the PRB innovation are minimal maintenance costs and long durability. However, the aim of this work is that future researchers will find a clear, in-depth and detailed explanation of groundwater contaminants, movement and detailed theoretical explanation for the fate of contaminants in the environment.
2. Groundwater Contamination
Groundwater is the global population’s main source of fresh water and is used for domestic, food production and industrial purposes. About a third of the world’s population depends on groundwater as the main water source for their drinking purposes [ 10 ]. According to the United Nations Environmental Agency (UNEP), there are 32 cities around the world with a population greater than 10 million known as “megacities”; about 16 of these cities majorly rely upon groundwater [ 8 ]. In China, there are 657 cities, and approximately 400 cities are using water from the ground as the main source for their water supplies [ 11 ]. It is without doubt that subsurface water/groundwater is an essential resource of water to humanity; furthermore, it is vital for the ecological system on earth. Keeping this water resource sustainable, accessible, effective and efficient is a major concern for scientists working in a related field. However, urbanization, farming, industry and climate change all pose significant threats to the quality of groundwater. Toxic metal, hydrocarbons, contaminants such as organic trace pollutants, pharmaceutical pollutants, pesticides and other contaminants are endangering human health, natural ecosystems and long-term socioeconomic development [ 12 , 13 ]. Chemical contamination has been a major subject in groundwater investigations in recent decades. While groundwater contamination poses a significant threat to human populations, it also provides a chance for researchers to learn more about how our underground aquifers have evolved, as well as for decision makers to understand how we might maintain the quality and quantity of these resources [ 14 ]. According to the Canadian government, the contamination of groundwater can be defined as the addition of undesired substances by human activities [ 15 ]. Chemicals, brines, microbes, viral infections, medications, fertilizers and petroleum can all contribute to groundwater contamination. However, groundwater contamination is differs from surface water contamination in that it is unseen, and recovery of the resource is difficult and expensive at the current technological level [ 16 ].
Due to human and natural activities, chemicals and pollutants may be found in groundwater. Metals such as arsenic, cadmium and iron could be dissolved in groundwater and may be found in high concentrations. Human activities such as industrial discharges, waste disposal and agriculture activities are the main cause of groundwater contamination. Furthermore, it could happen due to urban activities such as the excessive use of fertilizers, pesticides and chemicals in which pollutants migrate to groundwater and reach the water table. In any case, using groundwater for drinking, irrigation or industrial purposes requires different tests to ensure that it is suitable for these purposes.
The presence of inorganic contaminants in groundwater is a big concern especially when groundwater is used for drinking or agricultural purposes. If these contaminants are presented in the groundwater with levels higher than the permissible recommended concentration, they cause health problems throughout the food chain [ 17 ]. Table 1 presents different inorganic pollutants in groundwater, sources and health effects.
Inorganic pollutants presented in groundwater.
In addition, discharging organic pollutants into the environment and water resources represents a pressing concern for people’s health. The existence of organic contaminants in groundwater represents a crucial environmental problem, as it may affect the water supply reservoirs and people’s health [ 18 ]. Additionally, it can affect the ecological system [ 19 ]. Usually, groundwater contaminants come from two sources: (1) landfills, solid waste disposal lands, sewer leakage and storage tanks leakage and (2) agriculture and farmyard drainage [ 20 ]. Table 2 shows the most common organic pollutants usually found in the groundwater, the sources and the health effect.
Organic contaminants, source to groundwater and their effects.
In the environment, groundwater in shallow or deep aquifers is never found completely sterile [ 21 ]. Coliform organisms and bacteria are the main cause of the microbiological pollution of groundwater. When present, these pollutants need immediate attention to protect lives from outbreaks of pathogenic disease [ 22 ]. Microbiological contaminants naturally occur in the environment by the intestines of humans, warm-blooded animals and plants. These microorganisms could cause dysentery, typhoid fever and different diseases [ 21 ].
3. Groundwater Treatment Technologies
In recent decades, scientists developed sophisticated and highly successful techniques for the remediation of water from many contaminants. These techniques generally focused on the treatment of surface water resources such as a river, lakes and water reservoirs. However, in recent years, scientists and environmental researchers have become more aware of treating underground water, and groundwater has become an essential source of water in most places; it represents about 30% of the freshwater reserve in the world [ 29 , 32 , 37 , 38 ]. Groundwater is usually treated by drilling water wells, pumping the polluted water to ground facilities to perform different approaches of treatment such as air stripping and treatment tower and granular activated carbon (GAC). Pressurized air bubbles are also used to treat contaminated groundwater. The selection of the effective treatment/remediation procedure depends on the characteristics of contaminants and pollutants, in addition to the reactive media available [ 39 ].
3.1. Pump and Treat Method
One of the popular procedures to remediate contaminated groundwater is by dissolved chemicals, solvents, metals and fuel oil [ 40 ]. In this procedure, contaminated groundwater is piped to ground lagoons or directly to treatment units, which treat the groundwater using various methods such as activated carbon or air stripping. Finally, the treated water is to be discharged either to the nearest sewer system or re-pumped to the subsurface [ 37 ]. This technique can treat large volumes of contaminated groundwater but has many disadvantages, such as the high cost, spreading of contaminants into the ecosystem, as well as its long operation time; in addition, it may cause a reversal to the hydraulic gradient [ 41 , 42 , 43 ] as cited in [ 40 ].
3.2. Air Sparging Procedure and Soil Vapor Extraction
The procedure of air sparging and soil vapor extraction (SVE) is considered one of the most common techniques used in remediating groundwater contaminated by volatile organic contaminants (VOCs). It is considered efficient, fast and relatively economical [ 44 ]. This method involves the injection of pressurized air at the lowest point of the contaminated groundwater; this will clean up the groundwater by changing the state of volatile hydrocarbons to a vapor state. While pumping air under the saturated zone, pollutants are stripped out of the aquifer and oxygen is provided for the biodegradation of contaminants [ 45 ]. The extracted air is to be treated by vacuum extraction systems to remove any toxic contaminants [ 46 ]. The limitations for this method are the high cost when working in hard surface area and when many deep wells are required for the treatment. In addition, soil heterogeneity may lead to uneven treatment of the contaminated groundwater.
3.3. The Permeable Reactive Barriers (PRBs)
It is an innovative remediation technique [ 47 ]. Practically, it is in situ technology to remediate groundwater using reactive media designed to intercept a contaminated plume. Typically, reactive media is designed to degrade volatile organics, immobilizing metals. PRB media is placed with porous materials such as sand; this will enhance the hydraulic conductivity, so the plume of contaminants will pass through the PRB under a natural gradient descent [ 37 , 48 ].
In the treatment wall, contaminants are removed by adsorbing, transforming, degrading and precipitating the targeted pollutants during water flow through barrier trenches. PRBs are defined as an in situ remediation zone in which contaminants are passively captured, removed or broken down while it allows uncontaminated water to pass through. The primary removal method is either physical (sorption, precipitation), chemical (ion exchange) or biological [ 49 , 50 , 51 , 52 ].
There are many geometries for placing the permeable reactive barriers (PRBs): (1) A continuous wall that contains reactive media. This is the most common placement in which the reactive media is placed perpendicular to the contaminated plume of groundwater flow; (2) funnel and gate in which contaminant plume is directed to a treatment filtering gate by two-sided impermeable walls at sites in which the soil is very heterogeneous, placing the PRB in the most permeable portion of the soil. Furthermore, when the contaminant’s distribution is non-uniform, the pollutant’s concentration can be better homogenized when entering the PRB gate; (3) radial filtration/caisson configuration in which the filter is placed in a cylindrical shape of reactive media surrounded by coarse material with a core of course materials. Additionally, there must be a radial centripetal flow by applying a hydraulic gradient. The third type of PRB has a long lifespan and a better treatment efficiency by extending the contact time between the pollutant and the reactive barrier [ 47 , 53 , 54 ].
Different reactive materials can be used to remediate contaminants, for example, zero-valent iron (ZVI; Fe0), which is a mild reductant and can treat heavy metals. ZVI can de-halogenate may halogenate hydrocarbon derivatives [ 55 ]. Bio-sparging materials and slow oxygen releasing compounds have the ability to treat groundwater containing petroleum hydrocarbon plums such as nitrobenzene and aniline by utilizing the biodegradation of these pollutants in PRBs [ 56 ]. Vegetative materials could be used in PRBs such as mulch to remediate chlorinated solvents and perchlorates [ 57 ].
Contaminants can also be precipitated on chemical reactive materials in the PRBs, for example, fly ash, ferrous slats, lime, phosphates and zeolites, iron/sand, iron/gravel, iron/sponge, granular activated carbon, organic carbon, copper wool and steel wool [ 37 , 54 ].
3.3.1. Characteristics of the Reactive Medias
Choosing a good reactive media depends on the following characteristics [ 58 ]:
- 1. Reactivity: The ability of reactive media to react/remediate contaminants and the equilibrium constant. All these factors are necessary to determine the required time for the remediation, which is important to calculate the volume and size of the in situ reactive barriers.
- 2. Stability: It is required that any good reactive material is to be active for a long period to remediate groundwater. Additionally, it is also necessary that the reactive media stay under the surface as a secondary precipitate. Once the PRB is installed, it is very expensive to be excavated and replaced with a new PRB.
- 3. Cost and availability: it is very important that the reactive media be available and inexpensive.
- 4. Hydraulic conductivity: the PRB must have a permeability equal to or greater than the surrounding soil to ease the groundwater flow within the PRB and achieve the remediation.
- 5. Environmental compatibility: Reactive media need to be similar/match the surrounding subsurface soil by mean of grain size for the goal that there will be no change in the hydraulic conductivity of the soil. Additionally, it needs no unwanted by-products to be produced during the remediation.
3.3.2. Uptake Mechanism of Contaminants
In the remediation of groundwater from contaminants, four physical, chemical and biological uptake mechanisms are considered as uptake mechanisms [ 58 , 59 , 60 ], which are: (1) adsorption and ion exchange, (2) abiotic redaction, (3) biotic reduction and (4) chemical precipitation. Remediation of contaminants in groundwater can be achieved by two or more of these mechanisms [ 61 ].
- (1) Adsorption and Ion Exchange
The process in which species in an aqueous environment are attached to a solid surface is referred to as adsorption. Usually, adsorption interaction is considered a rapid and reversible phenomenon. Adsorbents such as zero-valent iron (ZVI), zeolite and amorphous ferric oxyhydroxide (AFO) are the most common adsorbents used in the adsorption of contaminants; most of the adsorbents have a large surface area per gram and could be used in a PRB. ZVI has the most adsorption rate, and it is the most popular reactive media used in PRBs. Adhesion of pollutant’s ions, atoms or molecules while it is in a liquid, gas or dissolved solid state is referred to as adsorption. It utilizes chemical forces to create a thin film of the adsorbate on the adsorbent’s surface. The adsorbent is any kind of material that can adsorb substances through its surface area characteristics. In the adsorption theory, the surface area of the adsorbent is predominant. The solid phase that provides a working adsorption area is the adsorbent, while the substances and species adsorbed on the adsorbent are referred to as the adsorbate. Adsorption efficiency depends on adsorbate concentration, liquid-phase temperature and pH [ 62 ].
Ion exchange is a process of remediation of inorganic chemicals and dissolved metals from liquids and groundwater. The ion exchange process is that the ion (a single atom or group of atoms) is either positively charged after its loss of electrons or negatively charged after gaining an electron. When liquids loaded by pollutants pass through the ion exchange resin, contaminated substances will be exchanged by the effect of metallic ions attraction by the resins. These resins can be re-generated after being exhausted, or it may be a single-use resin [ 63 , 64 ]. Ion exchange phenomena is a reversible reaction process in which a pollutant’s ion is replaced with an identical ion on the immobilizing barrier. Most ion exchangers are natural such as zeolite, but also, there are very good synthesized ion exchanger resins that can be used in specific needs, especially for the treatment of inorganic contaminants [ 58 , 60 ]. The ion exchange method is applicable to remediate heavy metals [ 65 ] and dissolved metals (chromium) from polluted liquids. Additionally, this method could be used to treat non-metallic pollutants such as nitrate and ammonia [ 63 ]. The limitation to the use of this method is that the oxidation of the soil will cause damage to the resin and will decrease remediation efficiency [ 66 , 67 ]. Another concern is that the contaminant has not been destroyed if treated by the ion exchange method; it is only transferred to another medium that needs to be disposed of. This method is not good if the groundwater contains oil or grease, as these pollutants may clog the exchange resin [ 67 ].
- (2) Abiotic Reduction
The chemical reactions that lead to the decomposition of contaminants in groundwater are referred to as abiotic remediation. In this technique, the harmful compounds are to be reduced either by immobilization in the treatment wall of the reactive barriers, or it is permitted to pass through the barrier in a harmless form. Zero-valent iron (ZVI) is the most popular reactive material used in the abiotic remediation of groundwater; after the reaction of ZVI with the contaminants, low solubility minerals will be precipitated, for example, the remediation of U and Cr from groundwater, which is removed by the precipitation of these contaminants by the abiotic process. Equation (1) shows the ability of ZVI to reduce U(VI) to U(IV) in groundwater with high carbonate and moderate pH via producing UO 2 (Uraninite), which is a solid, less crystalline product of uranium.
For the chromium (Cr), ZVI reducing Cr(VI) to Cr(IV) [ 58 , 60 ] as shown in Equation (2):
Cr(VI) could be reduced to Cr(III) by ferrous iron via introducing dissolved dithionite ions ( S 2 O 4 2 − ) to an aquifer, which can reduce the solid phase of ferric iron. Dithionite oxidizes to sulphite ( S O 3 2 − ) and F e 3 + is lowered to F e 2 + . Cr(III) is to be stalemated by precipitate in the solid form of Cr(III) and Fe(III) hydroxide along with the reduction in some halogenated organic compounds by the effect of F e 2 + as shown in Equations (3) and (4).
- (3) Biotic Redaction/Oxidation
When physical or chemical remediation of groundwater shows little or no degradation of contaminants, then degrading pollutants with a biological oxidation process may be helpful. Many pollutants such as chlorinated solvents tend to be easily reduced if oxidized; here, microorganisms will perform a reduction process by exploiting contaminants as their main source for energy and the required materials to synthesize their cells [ 49 ]. The bioremediation technique is a very effective remediation process based upon the degradation of contaminants by microorganisms; remediation efficiency in this process depends on the working environment, such as the temperature, pH, electron acceptors and the concentration of nutrients [ 68 ]. In biodegradation, it is necessary that germs use electron acceptors to accept any electrons liberated from pollutants; electrons transfer, releasing energy that is essential for microbes’ lives. In the presence of oxygen, under aerobic conditions (which is preferable), energy producing from this process is higher than that released without the presence of oxygen. Additionally, the oxidation rate of contaminants is higher. In the groundwater, the presence of oxygen is usually little; in this case, the anaerobic microbes electron acceptors is utilized. However, it is effective to remediate groundwater contaminated by monoaromatic hydrocarbons by using oxygen-releasing compounds in the PRBs [ 49 , 56 , 69 ].
The basic concept of biotic reduction, biotic oxidation, is to supply an electron donor along with nutrient materials to be used by microorganisms to break down the contaminants. Leaf mulch, wheat straw and sawdust can be used as electron donors, and municipal waste can be used as a nutrient material. Dissolved sulphate in the wastewater is a good electron acceptor, which can oxidate organic materials and can consume acidity coupling with metal reduction as shown in the below Equations (5) and (6):
- (4) Chemical Precipitation
This process consists of contaminants removal as hydroxides (Equation (7)) and carbonates (Equation (8)) via mineral precipitation resulting from increased pH. Firstly, contaminants are reduced to a less soluble species, and finally, they are retained as minerals in the barrier. Limestone (CaCo 3 ) and apatite [Ca 5 (PO 4 ) 3 (OH)] are commonly used in chemical precipitation
A summary of the available and common reactive media is presented in Table 3 ; the geochemical process, nature of contaminants, reactivity and availability are significant factors in the selection of the best convenient reactive media in remediating groundwater.
Reactive media for the remediation of groundwater contaminated by metals and radionuclides (Bronstein, 2005).
4. Modelling of Sorption Process
“Sorption” refers to the physical or chemical process in which a substance becomes in contact with another, which consists of two processes:
- (1) “Adsorption” is a surface process; substances transfer from their aqueous phase (liquid or gas) to the solid phase surface that provides a surface for adsorption known as “adsorbent”; the species transformed from the aqueous phase to the surface of the solid phase is called “adsorbate” [ 62 ]. The existence of nitro groups on the adsorbate stimulating adsorption, hydroxyl, azo groups increases the adsorption rate, while the presence of sulfonic acid groups decreases adsorption [ 70 ].
- (2) “Absorption” is defined as the whole transfer of substances from one phase to another without forces being applied to the molecules. The relationship governing the transfer of substances in aqueous porous media and the mobility of substances from liquid or gas states to the solid state is referred to as “isotherm” [ 71 ]. Adsorption isotherms is curvy relationships connecting the equilibrium concentration of a solute on the surface of an adsorbent ( q e ) to the concentration of solute in its aqueous state ( C e ); both phases should be in contact with each other [ 70 , 72 ].
4.1. Sorption Isotherm Models
Several isotherm models are used to describe sorption parameters and the adsorption of pollutants as follows:
4.1.1. Freundlich Model
In 1909, Freundlich gave an imperial relationship that describes the capability of a unit mass of solid to adsorb gas in the presence of pressure. The Freundlich adsorption isotherm is a curve correlation between a solute concentration on a solid’s interface and the solute concentration in the adjacent aqueous environment [ 73 ]. The Freundlich isotherm model describes absorption in the terms of adsorbate concentration as follows:
where K f m g g is the coefficient of the Freundlich isotherm, n < 1, which describes the empirical coefficient expresses the amount of sorption [ 72 , 74 , 75 ]. ( K f ) a n d (n) can be calculated by solving equation xx logarithmically and plotting ln q e verses ln C e where K f = 10 y − i n t e r c e p t and the slop of ( 1 n ) as shown below:
According to the Freundlich isotherm, the sorbet contaminants is directly proportional to their concentration at a small amount and decreases when contaminants accumulate at the surface of the reactive media [ 76 ].
4.1.2. Langmuir Model
The theoretical Langmuir isotherm model has been derived to describe the physical besides the chemical adsorption, as well as quantifying and describing the sorption on sites located on the adsorbent. Langmuir assumes the following [ 70 , 71 , 76 ]:
- Each adsorbate molecule is to be adsorbed on a well-defined binding site on the adsorbent, and adsorption reaches saturation when all these sites are occupied.
- Each active binding site on the adsorbent interacts with one adsorbate molecule only.
- No interaction existed between adsorbed molecules. All sites are homogeneous (energetically equivalents).
- The surface is uniform, and monolayer adsorption occurs.
Accordingly, the equation of the Langmuir isotherm model is:
where C e (mg/L) represents the concentration of solute in the bulk solution at the equilibrium state. q m (mg/g) represents the maximum adsorption capacity. b is a constant that represents sorption free energy. q e (mg/g) represents the amount of the adsorbed solute by a unit weight of adsorbent within the equilibrium conditions. The Langmuir equation’s constant can be determined with the linearization of Equation (12) as follows:
This equation describes that C e q e is plotted as a function of C e , the parameters of q m and b are determined from the slope ( 1 q m ) with y-intercept ( 1 q m b ) linear regression to Equation (12) [ 76 ].
4.1.3. Temkin Model
The Temkin isotherm assumes that heats of adsorption would more often decrease than increase with the increase in solid surface coverage. It takes into account the adsorbing species–adsorbent interaction. Temkin isotherm has the following formula:
where R represents gas universal constants (8.314 J/mol K). T is the absolute temperature (K). a T e and b T e are constants.
4.1.4. Brunauer–Emmett–Teller (BET) Model
The BET was developed based on the Langmuir model in an attempt to minimize the Langmuir isotherm restrictions. This isotherm assumes that more molecules can be adsorbed on the monolayer, and it is possible within this isotherm that bi-layer (multi-layer) adsorption will occur. This isotherm could be proclaimed as:
where q m is the maximum adoption capacity, b represents a dimensionless constant, and C s is the concentration in the case of saturated sites and homogenous surfaces.
4.2. Kinetic Models
Adsorption kinetic models are important to describe the solution uptake rate and adsorption required time [ 74 , 75 , 77 ]; these models providing a description for the sorption process onto the sorbents. The sorption mechanism occurs in three steps; the first one is the diffusion of adsorbate through the aqueous phase surrounding the adsorbent; secondly, the diffusion of adsorbate in the pore of the particle (intrapore diffusion); finally, the adsorption occurrence due to physical or chemical interaction between the adsorbate and adsorbent [ 75 , 78 , 79 ]. However, three kinetic models are used to describe the sorption mechanism and the predominated stage as follows:
4.2.1. Pseudo-First-Order Model
A model that is quantified according to Equation (15) below:
where q e is the contaminant’s amount sorbet in equilibrium conditions (mg/g), q t represents a contaminant’s quantity sorbet during any given time (t) (mg/g), k 1 is a constant rate of pseudo-first-order adsorption (min −1 ).
The pseudo-first-order equation has been integrated at boundary conditions of t = 0 to t = t and q t = 0 to q t = q e , then transferred to a linear form as shown in Equations (16) and (17) [ 80 ].
For this kinetic model, log q e − q t must be plotted against time interval; if the intercept of q e theoretical differs than q e experimental , then the reaction does not follow the model of the pseudo first order.
4.2.2. Pseudo-Second-Order Model
The kinetic model of pseudo-second-order adsorption is applicable for small initial concentrations to calculate the initial sorption rate [ 74 ]. The pseudo-second-order equation for the sorption rate has the following form:
where q t is the magnitude of adsorbate, which is adsorbed by an adsorbent (mg g −1 ) at a given time (min), q e represents the amount of adsorbate adsorbed (mg g −1 ) in equilibrium conditions. k 2 is a constant of the second-order sorption rate (mg (mg min) −1 ) [ 80 ].
4.2.3. Intra-Particle Diffusion Model
In 1962, Weber and Morris proposed the kinetic model of intra-particle diffusion, and it has been used for the analysis of adsorption kinetics of lead ions by adsorbent (CHAP) [ 76 , 80 , 81 ]. Based on this model, the uptake graph of ( q t ) versus the squared root of time ( t 0.5 ) must be linear in the overall adsorption process; in addition, if the line intersects with the origin, then the intra-particle diffusion is the predominant adsorption process. The k d represents the intra-particle diffusion initial rate (mg (mg min) −1 ), which could be calculated through the following formula:
where q t represents the amount of sorbate on the solid phase (surface of sorbent) at any time t (mg g −1 ), and t represents time (min).
5. Contaminant Transport Equation and Breakthrough Curves
Soil is a dynamic system in which toxic contaminants are used as a sink or a pathway. When contamination occurs on the surface soil, some of these contaminants will percolate under the water table and form a plume of contaminants. This plume will be developed over time ( t ), and contaminants will be driven downstream, as shown in Figure 1 . It is very important to understand how these contaminants will dissolve in the flow and how they will be carried out downstream; it is very important to discover the concentration of these contaminants as a function of time. The predominant mechanism for the attenuation and retardation of contaminants is sorption. Sorption phenomena will happen when the solid phase of the environment attenuate these contaminants, which will lead to contaminants being removed from the water, and the concentration of pollutants will be reduced downstream. The transport mechanism of pollutants in a saturated environment is the advection that carries contaminants without mixing. The hydrodynamic dispersion is driven by molecular diffusion and mechanical dispersion. If the hydraulic dispersion goes to zero, then the transport will be conservative, and there will be no retardation or any attenuation to the contaminants; on the contrary, if there is retardation to the contaminates, then the concentration of contaminants will be reduced at the downstream by the effect of sorption.

Contaminants concentration development in groundwater (“t1, t2–t6” are time intervals).
5.1. Modeling of Contaminants Transport
5.1.1. advection.
In the advection, contaminants transport downstream along with the flow with advective velocity. It is the physical transport of contaminants across the space:
where V x a is the linear advective velocity.
Darcy velocity is given by the meaning of Darcy law, which is:
where ( K ) is the hydraulic conductivity, ( K r ) is the relative conductivity, ( θ ) is the volumetric moisture content, and ( ∂ h ∂ x ) is the head gradient in the x-direction.
where (F x ) is the advective flux ( K g t . m 2 ), ( V x a ) is the advective liner velocity ( m s e c ), ( n ) is the effective porosity, and ( c ) is the concentration of contaminants ( k g m 3 )
Substitute F x , F y and F z in the conservative equation:
In the saturated medium, ( n ) = 1.
5.1.2. Hydrodynamic Dispersion
Molecular diffusion.
In a stagnant fluid, diffusion is the process of molecules random movement. It is basically driven by the concentration gradient and occurs by the Brownian motion. Therefore, diffusion usually increases with the increment of entropy.
In general, diffusion follows Fick’s first law:
where ( F ) is the mass of solute per unit area per unit time ( M L 2 T ), ( D d ) is the diffusion coefficient ( L 2 T ) ≈ 10 − 9 ( m 2 s e c ), and ( ∂ c ∂ x ) is the concentration gradient ( M L 3 L ).
According to the mass conservation of dissolved contaminants:
The time-dependent concentration equation is:
n = 1 in a saturated medium.
Substituting Fick’s first law in Equation (28)
For a one dimensional flow:
The diffusion coefficient ( D d ) here is the free diffusion coefficient (i.e., in water); if the flow medium is porous, then the effective diffusion coefficient ( D * ) is used due to the effect of the tortuous flow path:
w is related to the tortuosity (T): T = l e l ≥ 1 as shown in the below Figure 2 ; laboratory studies showed that 0.01 > w ≤ 0.5

Determination of tortuosity in a porous medium.
Mechanical Dispersion
There is a number of mechanisms that lead to the assurance of the mechanical mixing of contaminants in the aquifer as follows:
- (a) Mechanical dispersion due to pore size
When dissolved contaminants pass through a porous medium, pore size will affect the hydraulic conductivity of this media; when particles are fine, porosity will be below, and the advective velocity will be slow, as shown in Figure 3 .

Mechanical dispersion due to pore size.
- (b) Mechanical dispersion due to path length
If a pore is medium, the mechanical mixing may happen due to the effect of the length of the pathway, which will be passed by the dissolved contaminants. Each molecule of contaminants will pass through a different pathway that is unequal with the pathway of other particles, as illustrated in the below Figure 4 .

Mechanical dispersion due to path length.
- (c) Tylor dispersion
Taylor mechanical dispersion occurs when dissolved contaminants pass around the aquifer’s solid particles. Solids pass faster in a middle way between two particles than another pass near a solid particle, as shown in Figure 5 . This is because the linear velocity in the centre of pores is greater than that near the edge of solid particles.

Tylor mechanical dispersion.
All the above mechanisms lead to mechanical mixing for solute contaminants in both the longitudinal direction (with the main flow direction) and the transverse direction (out of the main flow direction).
The coefficient of mechanical dispersion ( D ) is related to aquifers’ dispersivity (α), which reflects the extent to which the aquifer is dispersive and the advective velocity of flow.
where ( D L ) and ( D T ) are the mechanical dispersion coefficient in the longitudinal and transverse directions (m 2 /sec), respectively. ( α L ) and ( α T ) are the longitudinal and transverse dispersivity (m), respectively. ( V L a ) and ( V T a ) are the longitudinal and transverse advective velocity (m/sec).
In the low permeability medium, the permeability is near to zero; in this case, there will be no effect on the mechanical dispersion, and only the diffusion will be predominant.
5.1.3. Advection–Diffusion Equation
The theory of contaminants transport model in porous media is subjected to a partial differential equation governing space and time. The theory incorporates four different processes, all merged in one equation; one process is advection, which means that a substance follows the direction of water (driven by water flow) and itself moves with convection. The second process is dispersion, which is caused by the heterogeneity of pollutants, and a package of contaminants will move faster than the others. Then, there is a chemical reaction, which described by a kinetic equation. Finally, there is the adsorption to the soil, which means that the contaminant may spend some of its time tied to the solid phase and sometimes in the mobile water. The equation that describes all of this is the advection–dispersion equation, as follows:
In the above equation, the change in mass per unit volume ( m ) of the contaminants due to the reactions within the aquifer is referred to as ( r ).
where ( F x ) is the total flux in the ( X ) direction. ( V x n C ) is the addictive flux, and (– ( n D x ∂ C ∂ x )) is the dispersive flux.
Substituting ( F x ) in Equation (37) for (x, y and z) directions:
In the 1D flow, with a constant dispersion coefficient and constants porosity in space and time (=1 in a saturated medium), the equation of advection–dispersion can be written as:
The term ( r ) is considered an important factor in the attenuation of contaminants in a porous media, which is related to the sorption, the predominant process of contaminants attenuation in a permeable reactive barrier during contaminants’ mass transfer. Generally, ( r ) depends on the bulk density ( ρ b ) of the medium and the amount of contaminants sorbed ( q ) with time, thus:
By substituting the value of ( r ) (Equation (41)) in Equation (40), the advection–dispersion will be as follows:
The sorption process is represented in the above equation by the term ρ b n ∂ q ∂ t , ( q ) represents contaminants concentration that sorbed on the solid phase of the reactive media, which can be described by the Langmuir or Freundlich isotherm models as a function of concentration. Equation (42) can be rewritten as follows:
where ( R ) is the retardation factor, which reflects the effect of retardation of contaminants during its transport to the downstream.
The “breakthrough curve” describes the relationship between the concentration of contaminant vs. time, which is an important tool for design and optimizes the sorption in a field-scale PRB by relating the data obtained from laboratory columns to the field scale breakthrough curves. In a continuous constant influent of contaminants, the breakthrough curve will be shaped as (S); the best point on this curve is referred to as the breakthrough point, which has an outlet concentration of contaminants that matches the desired concentration in water. A summary of empirical and theoretical models used to predict the breakthrough curves are described below:
- Bohart–Adams model
The purpose of performing column experiments is to calculate the relationship between the concentration and time, the breakthrough curve in addition to calculate the maximum adsorbent capacity of adsorption. Results will be used to design a full-scale adsorption column. The Bohart–Adams model is one of the models that has been formulated to fulfil this purpose; it has been based on the rate of surface reaction theory [ 82 ]. This model has been built on the following assumptions [ 48 ]:
- 1. This model can describe the concentration at low levels ( C « C 0 ) ( C = 0.15 C 0 ).
- 2. When t → ∞ ; q 0 → N 0 with saturation concentration.
- 3. The external mass transfer is limiting adsorption speed.
- 4. The Bohart–Adams model has the following formula: C C 0 = 1 1 + exp K N 0 Z U − K C 0 t (44) where C 0 and C represents the instantaneous (initial) concentration of the pollutant in solution (mg/L). K is the kinetic constant (L/g/min). N 0 represents the congestion concentration (mg/L). Z represents column bed depth (cm). t represents the time of service (min), and U is the velocity of the flow (cm/min).
- Thomas model.
The Thomas model is widely used to calculate adsorbent maximum adsorption capacity. It uses data obtained from continuous column experiments. The Thomas adsorption column is given below:
where C 0 and C are the concentrations of influent (mg/L). K T is the constant rate (mL/mg/min), q represents the higher adsorption capacity (mg/g), M represents an adsorbent quantity in the column (g), t is the time of adsorption (min), and Q is the feed flow rate (mL/min). The Thomas model is based on the following assumption:
- 1. No dispersion is driven.
- 2. The Langmuir isotherm coincide with the equilibrium state.
- 3. Adsorption kinetics ( K ) should follow the rate of pseudo-second-order law.
- Yoon–Nelson model
In this model, the decreasing probability of each adsorbate is proportional to its breakthrough adsorption on the adsorbent. The following formula is a representation of this model:
where K Y N represents the Yoon–Nelson rate constant. The Yoon–Nelson model is limited by its rough form.
- Clark Model
Clark’s breakthrough curves were based on the mass transfer principle in conjunction with the Freundlich isotherm. Clark has developed his breakthrough curves as follows:
where n represents the exponent of the Freundlich isotherm, A and r represents the parameters of the kinetic equation.
Wang et al. (2003) invented a new model based on the mass transfer model. It has been used as a solution of Co and Zn ions in a fixed bed under the following assumptions:
- 1. The adsorption mechanism is isothermal.
- 2. The mass transfer equation is as the following: − d y d t = K w x y (48) where K w represents the kinetic constant, the fraction of adsorbed metal ions is represented by y . (with x + y = 1 ) , x represents the fraction of metal ions moving through the fixed bed.
- 1. There is symmetry in the breakthrough curve.
- 2. The axial dispersion in the column is negligible.
By integrating the above equation and presuming that y = y w at t = t w . w = 0.5 , the entire breakthrough equation can be expressed as:
where ( x ) can be expressed as:
Finally, the Wand model, similar to the Yoon–Nelson model, cannot provide enough detail on the adsorption mechanism.
6. Review of Previous Research on the Use of PRBS
The first permeable reactive barrier was constructed at a Canadian air force base in (1991) [ 83 ]; since that date, many studies have been conducted to examine the PRB’s efficiency. There were 624 publications that discussed the permeable reactive barrier from 1999 to 2009 [ 84 , 85 ]. Previous research has been conducted to study the ability of different reactants to remediate different pollutants in the permeable reactive barrier. The following is a list of the most important scientific studies.
The remediation of groundwater contaminated by chlorinated ethenes such as vinyl chloride (VC), dichloroethene (DCE) and trichloroethene (TCE) was studied using in situ biodegradation with a special functional microorganism known as Burkholderia cepacia ENV435 [ 86 ]. The researchers chose these microorganisms for many important characteristics, such as their good adhesion ability to aquifers’ solids; in addition, these microorganisms can establish an organized existence without the need to induce co-substrates. Furthermore, these organisms can grow in a high density in fermenters (−100 g/L), and finally, they can accumulate high internal energy, which this microorganism can use to resist the effect of chlorinated solvents and survive. Results showed the concentrations of VC, DCE and TCE decreased by 78% after two days of organism injection.
The output of a pilot-scale PRB for the remediation of chlorinated volatile organic compound-contaminated groundwater (VOCs) has been investigated. This study used a granular zero-valent iron reactive barrier, which was mounted in a funnel with a gate mechanism. Results showed that consistent VOC degradation was observed over the research period. It is observed that the degradation mechanism is due to pH increment, which leads bicarbonate ( H C O 3 − ) to convert to carbonate ( C O 3 2 − ), the carbonate combines cations ( C a 2 + , F e 2 + , M g 2 + , etc . ) in solution, which form mineral precipitates. It is observed that mineral precipitates formed in the reactive media represented as an unconquerable limitation to the treatment process [ 87 ].
A zero-valent iron PRB’s effectiveness in eliminating chlorinated aliphatic hydrocarbons (CAHs) has been investigated. The contact of reactive media (ZVI) with the CAHs in an aqueous environment caused a rise in the pH; this resulted in the precipitation of carbonate minerals and a loss of 0.35% of the porosity in the reactive fraction of the PRB [ 88 ].
The rapid evolution of the PRB’s application from a full in situ implementation on a laboratory level to treat groundwater polluted by various types of inorganic and metals was assessed [ 89 ]. This study concluded that different reactive media can be used in the preamble reactive barrier to remove inorganic compounds, such as the use of zero-valent iron PRB to remove TC, U and Cr from groundwater. Furthermore, solid-state organic carbon may be used to extract dissolved solids associated with acid-mine drainage. According to this research, there are different mechanisms for the treatment of inorganic anions; for example, the rate of Cr(VI), TC (VII), U(VI) and NO 3 could be successfully decreased by the mean of reduction using zero-valent iron (Fe 0 ). According to a monitoring program for a Cr(VI)-contaminated area, the concentration of Cr(VI) has decreased from 8 mg L −1 to > 0.01 mg L −1 , owing to a decrease in Eh and an increase in pH.
At a former uranium production site in Monticello, Utah [ 90 ] investigated the design and efficiency of a PRB in extracting arsenic, uranium, selenium, vanadium, molybdenum and nitrate. In this study, field and laboratory column tests have been performed. The reactive media in PRB was the zero-valent iron. After one year from PRB installation, the performance of ZVI–PRB is described by the reduction in concentrations of elements up-gradient and down-gradient of the barrier. The inlet concentrations of arsenic, manganese, molybdenum, nitrate, selenium, uranium and vanadium were 10.3, 308, 62.8, 60.72, 18.2, 396 and 395 µg/L, respectively. These concentrations have reduced to be >0.2, 117, 17.5, >65.1, 0.1, >0.24 and 1.2 µg/L, respectively. The removal mechanism for these radionuclides is by reducing uranium to lower molecules along with precipitation. Additionally, adsorption is another chemical process that leads to a reduction in these elements.
The use of a reactive biological barrier to remove nitrate pollutants has been investigated. The autotrophic sulphur-oxidizing bacteria has been used as an electron donor, and sulphur granules have been used as a biological agent. Sulphur-oxidizing bacteria colonized the sulphur particles and removed nitrate, according to the findings. The best operation conditions have been investigated, and it was found that an environment near the neutral pH achieved 90% removal of nitrates [ 91 ].
The efficacy of a ZVI barrier mounted in the field in eliminating chromium solid-phase association has been studied, and the removal efficiency after 8 years of operation has been investigated. Results showed that ZVI has the ability to reduce the concentration of Cr from an average <1500 µg/L to about >1 µg/L. The reduction in Cr(VI) to Cr(III) along with the oxidation of Fe(0) to Fe(II) and Fe(III), resulting in Fe(III)-Cr(III) precipitating as oxyhydroxides and hydroxides, has been discovered to be the most common Cr removal mechanism. It was also discovered that the reacted iron produced a coating of goethite (α-FeOOH) with Cr, resulting in precipitation [ 92 ].
Experiments have been performed to discover the efficiency of seven selected organic substrates in removing inorganic nitrogen in the form of NO 3 − , NO 2 − and/or NH 4 + in a denitrification PRB in batch scale experiments. Softwood, hardwood, coniferous, mulch, willow, compost and leaves were all reactive materials. The softwood was found to be suitable for use as a reactive medium in PRB due to its very good ability to denitrify nitrogen. Reduction in nitrate was due to the effect of denitrification (which represents 90% of the nitrate removal of which the dissimilatory nitrate reduction to ammonia (DNRA) represents 10% of the removal process [ 93 ].
The efficacy of activated carbon PRB for removing cadmium from contaminated groundwater has been investigated. The original cadmium concentration was 0.020 mg/L, but after it passed through a PRB of activated carbon, the polluted plume was adsorbed, and the cadmium concentration was nearly zero for the first three months. After that, the barrier became saturated, but the effluent cadmium concentration remained below the quality limit of 0.005 mg/L for more than seven months [ 94 ].
The use of polyvinylpyrrolidone (PVP-K30)-modified nanoscale ZVI in removing tetracycline from liquid has been investigated. Tests revealed that PVP-nZVI consists of Fe(0) in the core and ferric oxides on the shell. PVP-nZVI will adsorb tetracycline and its degradation products, according to the findings. It is also observed that the adsorption of tetracycline has been reduced with time due to the formation of H 2 PO 4 − , which has a strong tendency to react with the mineral surface [ 95 ].
Tetracycline adsorption using graphene oxide (GO) as a reactive media has been investigated. Results showed that tetracycline formed a π–π interaction and cation–π bonds with the surface of GO, with the Langmuir and Temkin models providing the best fit isotherms for adsorption and the Langmuir model calculating a maximum adsorption capacity of 313 mg g −1 . The kinetics of the adsorption model are also equipped with a pseudo-second-order model with a better sorption constant ( k ), 0.065 g mg −1 h −1 than other adsorbents, according to the results [ 96 ].
The design, construction and testing of a permeable barrier at the Casey station in Antarctica to remediate and avoid the spread of an old diesel fuel spill have been discovered. Five segments of a bio-reactive barrier were allocated and installed in the funnel and gate configuration, each segment divided into three zones; the first one is a slow-release fertilizer zone to enhance the biodegradation, the second zone is responsible for hydrocarbon and nutrient capture and degradation, while the third zone is responsible for cation capture and access to nutrients produced by the first zone. The first zone’s reactive media was a nutrient source, followed by hydrocarbon sorption materials (granular activated carbon plus zeolite); to extract cations nutrient released and accessed from the first region, sodium activated clinoptilolite zeolite is used. Oxygen delivery to the system was applied to enhance the microbial reactions. The function of each zone is the first zone to provide nutrients such as phosphorate to the microorganism. Due to its high surface area and microporous surface (500–1500) m 2 /kg, granulated activated carbon can adsorb hydrocarbon pollutants in the second zone. In the third zone, the Australian sodium zeolite is placed to capture any accessed ammonium cation from the solution due to its high ability to exchange ions with ammonium. Tests and results showed that the ion exchange of zeolite best-controlled nutrient concentration, while the sodium zeolite captured any migrated ammonia from the groundwater. Additionally, results showed that the fuel is degraded in the PRB faster than in the hydrocarbon spill area field. In the cold world, activated carbon–PRB is a strong technology for removing hydrocarbons.
In batch and fixed-bed column experiments, the adsorption of tetracycline (TC) and chloramphenicol (CAP) was investigated by [ 97 ] using bamboo charcoal (BC) as a reactive medium. The predominant mechanism of TC and CAP adsorption on BC is π – π electron-donor–acceptor (EDA), cation–π bond in combination with H-bond interaction, while the hydrophobic and electrostatic interaction has a minor effect on the adsorption. Results showed that BC has a strong adsorption capacity to TC and CAP; with increasing influent concentration and flow rate, adsorption efficiency improves. Surface diffusion was the most common mass transfer mechanism for antibiotic adsorption [ 98 , 99 ].
An overview of the use of PRBs in the remediation of a broad range of pollutants, demonstrating that it is a viable alternative to the pump-and-treat process, has been discussed by [ 85 ]. The most popular PRB reactive media, according to this study, is zero-valent iron (ZVI). Efficient PRB architecture requires accurate site characterization, groundwater flow and flow conditions requirements and ground flow modelling.
The potential efficiency of a microscale zero-valent iron PRB in removing tetracycline (TC) and oxytetracycline (OTC) with the formation of transformation products during the remediation have been discovered. To investigate the effect of solution pH, a series of batch experiments were carried out, including iron dose and environment temperature. Results showed that pH has a key factor controlling the efficiency of removal; increasing iron dose and working temperature also increased the removal efficiency. Pseudo-second-order model and Langmuir isotherm were found to be most fitted to adsorption kinetics and removal isotherms [ 100 ].
The effectiveness of removing copper ions Cu(II) and zinc ions Zn (II) heavy metals from groundwater using cement kiln dust and a sand PRB was investigated by [ 48 ]. In this research, the re-use of a very fine by-product powder resulted from the cement industry known as cement kiln dust (CKD) has been investigated to remove appointed heavy metals instead of throwing this CKD into the environment. The optimum weight ratio of CKD/sand, which provides the best remediation, has been investigated in column tests from 99 days of operation time. The remediation mechanisms were the adsorption/desorption, precipitation/dissolution and adsorption/desorption of the pollutants. Contaminant transport in porous media, as well as breakthrough curves, are also explored. Breakthrough curves refer to the relationship between the concentration of the contaminants at any time in any position in the domain. Results showed that the best CKD/sand ratio was (10:90 and 20:80) because other ratios showed a loss in the hydraulic conductivity and loss in groundwater flow due to the accumulation of contaminants mass in the voids between the sand causing clogging and flow loss.
The mechanism of remediating pharmaceutical pollutants (tetracycline) from groundwater using zero-valent iron coupled with microorganisms as reactive media has been investigated by [ 55 ]. In this research, three PRB columns have been studied, beginning with columns filled by zero-valent iron, the second with zero-valent iron and microorganisms and, finally, the third one with microorganisms. Results revealed that zero-valent iron has the best effect on removing tetracycline. Removal efficiency reaches 50% while it was 40% with zero-valent iron and microorganisms’ PRB and 10% by the effect of microorganisms’ PRB. The mechanism of this reaction is that the zero-valent iron (Fe 0 ) has been adsorbed and reduced tetracycline, Fe 0 converted to Fe +2 and Fe +3 , and the tetracycline has been degraded.
The use of a bio-PRB coupled with a good aeration system to remediate groundwater polluted with nitrobenzene and aniline have been studied. To degrade the NB and AN, suspension-free cells of the degrading consortium and the immobilized consortium were used in this study. Results showed that both AN and NB were completely degraded within 3 days in the immobilized consortium, while it needs 3–5 days to degrade using the free cells. It was also discovered that in the presence of oxygen, the removal efficiency of NB and AN was increased [ 56 ].
In a permeable reactive barrier, [ 101 ] investigated the effect of MnO 2 and its mechanism of tetracycline elimination. The zero-valent iron serves as the reactive media in this PRB. In this research, three PRB columns were studied, the first one with ZVI, the second had ZVI-MnO 2 , while the third consisted of MnO 2 only. Results show that the ZVI in the presence of MnO 2 is the most effective material in removing TC. Its removal efficiency reached 85%, while the ZVI removed about 65% and the MnO 2 removed 50% of TC. This research revealed that MnO 2 accelerated the transformation of Fe 2+ to Fe 3+ , then the Fe 3+ degraded tetracycline. The functional group that played the predominant role in this reaction is the hydroxyl radical produced in this process.
A series of laboratory and field studies in the Ukrainian city of Zhovty Vody has been performed to assess the reliability of a reactive barrier made up of zero-valent iron and organic carbon mixtures to remediate uranium-contaminated groundwater. In these studies conducted by [ 102 ], three reactive media were examined. The first was zero-valent iron, which was used to study the sorption, reduction and precipitation of redox oxyanions; the second was the phosphorate materials, which has been used to transfer the dissolved materials to other phases; the third was bioremediation materials and organic carbon substrates. The study revealed that the treatment mechanism of the uranium is sorption by the ZV, and it also observed that the microbes have the ability to sorb the uranium U(VI) to the bacterial cell walls. Due to the effect of enzymatic production, dissolved oxygen reduced first, then due to the effect of denitrification, UO 2 CO 3 reduced to uranite and sulphate reduced to sulphide; finally, amorphous uranium oxide will be formed on the microorganism surfaces. In this research, new placement of the reactive media has been used in which rows of cylinders with iron reactive media have been placed instead of the regular funnel and gate placement; this placement reduced the in situ installation cost.
The effectivity of PRB made from sodium alginate/graphene oxide hydrogel beds (GSA) for the remediation of ciprofloxacin (CPX) antibiotic contaminating the groundwater has been investigated. In this research, the key factors affecting the performance have been studied, and longevity and the cost of PRB have been discussed, and a proper design for the PRB has been proposed. Results show that the adsorption capacity of CPX on the GSA was 100 mg for each gram of GSA at pH 7.0; the leading mechanism in the adsorption process was the pore filling, H-bonding, ion exchange, electrostatic interaction and hydrophobic interaction. The results indicate that the GSA’s ability to remove CPX from groundwater when used in a PRB is concrete evidence that GSA is a good option for removing CPX from groundwater [ 103 ].
The removal of tetracycline from aqueous solutions using binary nickel/nano zero-valent iron (NiFe) reactive media in column reactors has been studied. Results show that if a mixture of 20 mg/L of TC plus 120 mg/L of NiFe in a 90 min time of interaction, TC will be removed by 99.43%. In this research, sand particles loaded with reactive media (NiFe) have been used. Electrostatic interaction has been used to load the reactive media on sand particles. A Tc removal mechanism was investigated using UV-Visible spectroscopy, TOC, FTIR and SEM analysis [ 104 ].
The use of the PRB system in preventing the migration of radiocesium into groundwater using natural zeolite and sepiolite has been investigated. These reactive media are natural, low-cost materials. Two-dimensional bench-scale prototypes at the steady flow conditions have been used in the experiment. Information on the transport behaviour of radiocesium and changes in hydraulic conductivity were investigated in this study. It has been determined that the remediation phase would reduce hydraulic conductivity over time. As a result, by combining sand with reactive media, the PRB has been modified to achieve steady-state operating conditions of flow [ 105 ].
The effectivity of the use of PRB of cement kiln dust as a reactive media in an acidic environment (pH 3) to remediate groundwater contaminated with dissolved benzene has been studied by [ 9 ]. Experiments were performed for 60 days with batch and column tests. Results showed that benzine removing efficiency reached more than 90%, and the best CKD/sand ratio was 5/95, 10/90 and 15/85, which achieved the best hydraulic conductivity. Results also show that barrier longevity reached (half a year) when CKD was about 15%. FTIR test results showed that adsorption happened due to the formation of H bonding and cation.
The removal of meropenem antibiotic with a cement kiln dust (CKD) PRB through batch and continuous column experiments have been studied by [ 106 ]. Results showed that pH 7.0 had a 60 mg adsorption potential for every 1 g of CKD, according to the findings. Initial concentration, flow rate and influence have all had an impact on CKD efficiency. Meropenem adsorption occurred due to the O-containing functional group’s effect on the surface of CKD, which leads to an H-bonding and π – π a n d n – π EDA interaction (donor–acceptor) between the CKD and the meropenem, which all lead to the adsorption.
The sustained treatment of a bio-wall and its effectivity in remediating groundwater contaminated by chlorinated volatile organic compounds (TCE) after 10 years of bio-wall installation has been studied by [ 107 ]. The reactive medium used in this barrier was mulch, utilizing the benefit of its high cellulose content (<79%). This research investigates a reactive barrier of mulch (1615 m long × 10.7 m depth × 0.6 m thickness). This bio-barrier consisted of 42% mulch, 11% cotton, 32% sand and 15% rock to increase the permeability. It is estimated that groundwater retention time within the barrier is 2–50 days, while groundwater speed was (0.002–0.3 m/day). Contaminants were trichloroethene (TCE), tetrachloroethene (PCE), dichloroethene (DCE) and vinyl chloride (VC). After 10 years of the bio-wall installation, results showed that mulch bio-wall effectively degrades TCE from groundwater to daughter products, TCE concentrations remained below the USEPA maximum levels, while it was over these levels in the up-gradient side of the bio-wall. The microbial population, geochemical environment of the barrier was still active. Investigating the concentration patterns, microbial community and the geochemical environment of the bio-wall demonstrates that the bio-wall is an effective reductive to the volatile organic contaminants.
The effectiveness of a horizontal PRB with a reactive media of zero-valent iron to prevent the scattering of chlorinated solvent vapour in the unsaturated region was investigated by [ 108 ]. In this research, the potential feasibility of using PRBs placed in a horizontal direction was investigated. The reactive medium in this study was the zero-valent iron (ZVI) powder mixed with sand, and the TCE was tested as a model for the (VOCs). Tests were performed in batch reactors. Results showed after 3 weeks of treatment and based on the type of ZVI powder, the concentration of TCE vapour was reduced in a range of 35–99%. The ZVI’s best output is determined by the particular surface area.
The use of sewage sludge and cement kiln dust to produce hydroxyapatite nanoparticles has been investigated. The removal of tetracycline using the new formed hydroxyapatite were examined and the best operation conditions were 2 h contact time, dosage 0.4 g/50 mL, agitation speed 200 rpm with a mixture molar ratio Ca/P = 1.662, the removal efficiency reached 90% with a TC maximum adsorption capacity of 43.534 mg for each gram of hydroxyapatite filter cake. Results show that adding 10% sand (to enhance the hydraulic conductivity of the PRB) to the hydroxyapatite reduced the adsorption capacity to be 41.510 mg/g. XRD, FTIR and SEM analytical tests proved that the predominant mechanism for the remediation of TC is due to the adaptation on the hydroxyapatite surface. During the process, two functional groups, (-OPO3H-) and (CaOH2+), were formed, both of which are positively charged with the ammonium functional group and negatively charged with the phenolic diketone moiety of TC species. The removal of TC was also aided by the effect of hydrogen bonding and surface complexes formed between TC and Ca [ 109 ].
7. Conclusions and Perspective
In recent decades, there has been an increment in the dependence on groundwater as a major source of freshwater for daily human needs, but in many places, groundwater is being polluted by organic and inorganic contaminants. It is very important to remediate groundwater before use to prevent the spread of contaminants to the neighbour environment. Many techniques and reactive materials have been used in the remediation of contaminated groundwater; one of the most popular technologies is PRBs, which is considered an affordable technology. It allows the treatment of multiple pollutants if a multi-barrier is being used. In PRB technology, there is no adverse contamination that may happen, as contaminants will not be brought out to the surface. On the other hand, this technology may have some limitations, such as the difficulty of detailed site characterization required prior to the design of PRB, and only contaminants passing the PRB could be treated in addition to the limited field data for the longevity of the PRB, so the prospective tendency is to use new by-product materials to improve PRB performance. In this way, the environment will be saved by the disposal of these unwanted by-products and will be considered a (green) refreshment to the environment.
Groundwater contamination is now a global issue; solving this problem involves close coordination between scientists at universities and government agencies, as well as the industry and decision makers at all levels. The way ahead for solving this problem must include addressing the levels of groundwater contamination in different countries by using developed measures, techniques and policies. In addition, the variation of the influence of groundwater contamination in different countries must be well studied, including the effect on climatic regions and geological features. To study groundwater contamination in the future, groundwater scientists will need to adopt and apply new technologies such as artificial intelligence, “big data” analysis, drone surveys and molecular and stable isotope analysis technologies. Finally, governments, especially those with developing economies, need to invest more in groundwater and encourage researchers, training and research in this important, valuable field.
Author Contributions
O.A.-H. and K.H. organized the conceptualization of the idea and the methodology employed in this paper. Following that, E.L., T.M.Č., I.N., A.A.H.F. and N.A.-A. worked on the critical evaluation of the existing techniques. All authors have read and agreed to the published version of the manuscript.
This research received no external funding.
Institutional Review Board Statement
Not applicable.
Informed Consent Statement
Data availability statement, conflicts of interest.
The authors declare no conflict of interest.
Sample Availability
Publisher’s Note: MDPI stays neutral with regard to jurisdictional claims in published maps and institutional affiliations.
Advertisement
Groundwater quality assessment using water quality index (WQI) under GIS framework
- Original Article
- Open access
- Published: 12 February 2021
- Volume 11 , article number 46 , ( 2021 )
Cite this article
You have full access to this open access article
- Arjun Ram 1 ,
- S. K. Tiwari 2 ,
- H. K. Pandey 3 ,
- Abhishek Kumar Chaurasia 1 ,
- Supriya Singh 4 &
- Y. V. Singh 5
47k Accesses
129 Citations
Explore all metrics
Groundwater is an important source for drinking water supply in hard rock terrain of Bundelkhand massif particularly in District Mahoba, Uttar Pradesh, India. An attempt has been made in this work to understand the suitability of groundwater for human consumption. The parameters like pH, electrical conductivity, total dissolved solids, alkalinity , total hardness, calcium, magnesium, sodium, potassium, bicarbonate, sulfate, chloride, fluoride, nitrate, copper, manganese, silver, zinc, iron and nickel were analysed to estimate the groundwater quality. The water quality index (WQI) has been applied to categorize the water quality viz: excellent, good, poor, etc. which is quite useful to infer the quality of water to the people and policy makers in the concerned area. The WQI in the study area ranges from 4.75 to 115.93. The overall WQI in the study area indicates that the groundwater is safe and potable except few localized pockets in Charkhari and Jaitpur Blocks. The Hill-Piper Trilinear diagram reveals that the groundwater of the study area falls under Na + -Cl − , mixed Ca 2+ -Mg 2+ -Cl − and Ca 2+ - \({\text{HCO}}_{3}^{ - }\) types. The granite-gneiss contains orthoclase feldspar and biotite minerals which after weathering yields bicarbonate and chloride rich groundwater. The correlation matrix has been created and analysed to observe their significant impetus on the assessment of groundwater quality. The current study suggests that the groundwater of the area under deteriorated water quality needs treatment before consumption and also to be protected from the perils of geogenic/anthropogenic contamination.
Similar content being viewed by others
Evaluation of Groundwater Quality for Drinking Purposes Using the WQI and EWQI in Semi-Arid Regions in India
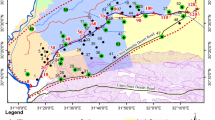
Water quality index for assessment of drinking groundwater purpose case study: area surrounding Ismailia Canal, Egypt
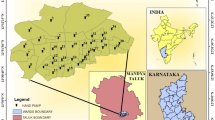
Groundwater quality evaluation using Water Quality Index (WQI) under GIS framework for Mandya City, Karnataka
Avoid common mistakes on your manuscript.
Introduction
In India, there has been a tremendous increase in the demand for groundwater due to rapid growth of population, accelerated pace of industrialization and urbanization (Yisa and Jimoh 2010 ). The availability and quality of groundwater are badly affected at an alarming rate due to anthropogenic activities viz. overexploitation and improper waste disposal (industrial, domestic and agricultural) to groundwater reservoirs (Panda and Sinha 1991; Kavitha et al. 2019a , 2019b ). Consequently, human health is seriously threatened by the prevailing agricultural practices particularly in relation to excessive application of fertilizers; unsanitary conditions and disposal of sewage into groundwater (Panigrahi et al. 2012 ). The groundwater quality also varies with depth of water, seasonal changes, leached dissolved salts and sub-surface environment (Gebrehiwot et al. 2011 ). According to the World Health Organization (WHO 2017 ), about 80% of all the diseases in human beings are water-borne. Once the groundwater is contaminated, it is difficult to ensure its restoration and proper quality by preventing the pollutants from the source. It, therefore, becomes imperative to monitor the quality of groundwater regularly, and to device ways and means to protect it from contamination. The quality of groundwater is deciphered using various physical, chemical and biological characteristics of water (Diersing and Nancy 2009 ; Panneerselvam et al. 2020a ). It is a measure of health and hygiene of groundwater concerning the need and purpose of human consumption (Johnson et al. 1997 ; Panneerselvam et al. 2020b ).
In recent years, the assessment and monitoring of groundwater quality on a regular basis is being carried out using Geographic Information System (GIS) technique added with the IDW interpolation method and has proved itself as a powerful tool for evaluating and analysing spatial information of water resources (Aravindan et al. 2010 ; Shankar et al. 2010 , 2011a , b ; Venkateswaran et al. 2012 ; Selvam et al. 2013b; Magesh and Elango 2019 ; Balamurugan et al. 2020b ; Soujanya Kamble et al. 2020 ). It is an economically feasible and time-efficient technique for transforming huge data sets to generate various spatial distribution maps and projections revealing trends, associations and sources of contaminants/pollutants. In this work, GIS technique has been used for spatial evaluation of various groundwater quality parameters.
In this study, the physicochemical properties of forty-three groundwater samples collected from wells and hand pumps were determined and compared with international standards of WHO for drinking and domestic uses based on Water Quality Index (WQI). The WQI was first developed by Horton ( 1965 ) based on weighted arithmetical calculation. A number of researchers (Brown et al. 1972 ; GEMS UNEP 2007; Kavitha and Elangovan 2010 ; Alobaidy et al. 2010 ; Shankar and Kawo 2019 ; Bawoke and Anteneh 2020 developed various WQI models based on weighing and rating of different water quality parameters which is derived by the weighted arithmetic method. The WQI is a dimensionless number with values ranking between 0 and 100. The WQI is a unique digital rating expression that expresses overall water quality status viz. excellent, good, poor, etc. at a certain space and time based on various water quality parameters. Thus, the WQI is being used as an important tool to compare the quality of groundwater and their management (Jagadeeswari and Ramesh 2012 ) in a particular region; and is helpful for selecting appropriate economically feasible treatment process to cope up with the concerned quality issues. It depicts the composite impact of different water quality parameters and communicates water quality information to the public and legislative policy-makers to shape strong policy and implement the water quality programs (Kalavathy et al. 2011 ) by the government.
Mineral intractions strongly influence groundwater hydrochemistry in aquifers and disintegration of minerals from various source rocks (Cerar and Urbanc 2013 ; Modibo Sidibé et al. 2019 ). Hydrochemistry of the analysed samples indicates that the mean abundance of major cations is present in order of Na ++ > Ca 2+ > Mg 2+ > K + while major anions in order of \({\text{HCO}}_{3}^{ - }\) > \({\text{NO}}_{3}^{ - }\) > Cl − > \({\text{SO}}_{4}^{2 - }\) > F − . The study shows that the sodium is dominant alkali while calcium and magnesium are the dominant alkaline earth metal leached in the aquafer due to rock water interaction affecting the quality of groundwater. Sodium in aquafer is derived from the weathering of halite and silicate minerals such as feldspar (Khan et al. 2014 ; Mostafa et al. 2017 ). The critical evaluation of Hill-Piper Trilinear diagram reflects Na + -Cl − , mixed Ca 2+ -Mg 2+ -Cl − , Ca 2+ - \({\text{HCO}}_{3}^{ - }\) , mixed Ca 2+ -Na + - \({\text{HCO}}_{3}^{ - }\) , Na + - \({\text{HCO}}_{3}^{ - }\) and Ca 2+ -Cl − type hydro-chemical facies in decreasing order of dominance. The Hydro-chemical characterization of groundwater reveals that the nature of aquifer is controlled by type of water, source and level of contamination (Aghazadeh et al. 2017 ; Brhane 2018 ). Hence, in order to keep the health of any aquaculture system, particularly an aquifer system at an optimal level, certain water quality indicators or parameters must be regularly monitored and controlled. Therefore, the objective of the study is to calculate the WQI of groundwater in order to assess its suitability for human consumption using the GIS interpolation technique and statistical approach in the study area.
Mahoba district is the south-western district of Uttar Pradesh which is adjacent to the state of Madhya Pradesh in south and Hamirpur (UP) in the north. The study area falls under the survey of India (SOI) toposheets no. 54O and 63C lies between latitude N25°01′30″ to N25°39′40″ and longitude E79°15′00″ to E80°10′30″ and covers an area of approximately 2933.59 km 2 . River Dhasan separates the district Mahoba from Jhansi in the west. A certainpart of Jhansi and Banda district has been merged in newly constructed Mahoba district in 1995 (bifurcated from Hamirpur). Mahoba district consists of three tehsils Kulpahar, Charkhari, Mahoba and four blocks Panwari, Jaitpur, Charkhari, Kabrai (Fig. 1 a). Kabrai is the biggest block fromaerial coverage as well as population point of view. Jaitpur is the smallest block from aerial coverage and Charkhari from population point of view. The study area experiences a typical subtropical climate punctuated by long and intense summer, with distinct seasons. The area receives an average annual precipitation of 864 mm mainly from the south-west monsoon. The temperature of the coldest month (January) is 8.3°C while the temperature of the hottest month (May) shoots upto 47.5°C. The entire area under investigation is characterised by highly jointed/fractured Bundelkhand granite (Archean age) with thin soil cover. Physiographically , the area is characterised by Bundelkhand massif terrain and is marked by the occurrence of solitary or clustered hillocks and intervening low relief with undulating plains. Two major physiographic units are: (1) Southern part having high relief with hillocks- This is south of 20°25′ N latitude & maximum altitude is 340 mamsl, reserved forest. Granitoids and intervening pegmatitic veins and numbers of quartz veins are observed. (2) Northern part relatively low relief with lower hillocks- In between 25°25′N and 25°39′N latitude and maximum altitude is 310 mamsl. The area in and around Panwari is mainly covered with thick alluvium, and hard rock is encountered only below 35 mbgl, coverage with seasonal forest. Pedi plain, pediment inselberg and buried pediplains are present.
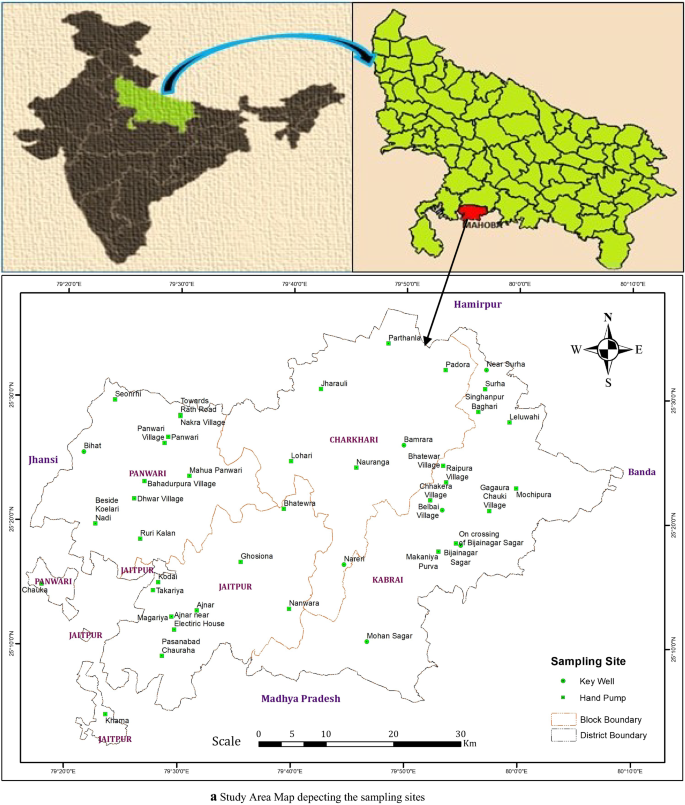
a Study area map depicting the sampling sites. b Geological map of study area
Geological and hydrogeological set-up
The granite, particularly leucogranite, older and younger alluvium consisting of clay, silt, sand and gravel mainly comprises the study area. The geological set-up of the study area indicates that the most dominant lithology is leucogranite covering mainly central and eastern part while recent alluvium covers the northern part (Fig. 1 b). At places, few patches of pink granite have also been recorded which appears enclosed in leucogranite or adjacent to its outcrop.The occurrence of groundwater is highly uncertain and unpredictable in this hilly and rugged terrain as it does not allow percolation and storages underground. The presence of porosity depends on the intensity of weathering and rock fracture which is responsible for groundwater occurrence, its quantity and flow mostly in permeable zones of weathered rock formations and under secondary porosity in the deep fractured zone. Groundwater recharge in the study area is triggered by the depth of overburden 7 m (Jaitpur-Kulpahar area) to 35 m (parts of Mahoba Tahsil and Charkhari block) as well as the intensity of weathering.
Materials and methods
The groundwater samples were collected during pre-monsoon (June 2016) period from the study area according to standard procedures of the American Public Health Association (APHA, 2017). The sampling locations were marked with the help of global positioning system (GPS) as shown in the Fig. 1 a. Samples were collected from the location through hand pump (depth: approx. 40 m) and dug wells (depth: 8–30 m ) as shown in Fig. 2 a–t. The collecting bottles (High-Density Polythene, HDPE) of one-litre capacity each were sterilized under the aseptic condition to avoid unpredictable contamination and subsequent changes in the characteristics of groundwater. Water samples were filtered using Whatman 42 filter paper (pore size 2.5 μm) prior to collection in the bottle. The sample was kept in the ice-box (portable) and brought to NABL accredited (ISO 17,025: 2017) laboratory of Central Ground Water Board (CGWB), Lucknow and Department of Soil Science & Agricultural Chemistry, Banaras Hindu University, Varanasi, UP, India. The samples were stored in a chemical laboratory at temperature 4–5 °C. The samples for metallic parameters were added 2 ml elemental grade nitric acid to obtain the pH 2–3 after acidification. The samples were pre-filtered in the laboratory to carry out the analysis. In the present study, a total of 20 groundwater quality parameters of forty-three samples were analysed as per test standard methods (APHA 2017) in the laboratory except for unstable parameters viz. hydrogen ion concentration (pH), electrical conductivity (EC) and total dissolved solids (TDS) which are determined by portable device (pH-meter, EC-meter and TDS-meter) in situ. Alkalinity (AK), Total hardness (TH), calcium (Ca 2+ ), magnesium (Mg 2+ ), bicarbonate ( \({\text{HCO}}_{3}^{ - }\) ) and chloride (Cl − ) were analysed using volumetric titrations; sodium (Na + ) and potassium (K + ) were analysed using systronics flame photometer model 129; nitrate ( \({\text{NO}}_{3}^{ - }\) ), fluoride (F − ), sulfate ( \({\text{SO}}_{4}^{2 - }\) ), were analysed using shimadzu 1800 spectrophotometer. Prior to analysis of the heavy metals viz. copper (Cu), manganese (Mn), silver (Ag), zinc (Zn), iron (Fe) and nickel (Ni); the groundwater samples were acidified with 1:1 nitric acid and concentrated ten times. The samples were subjected to analysis using Shimadzu 6701 Atomic Absorption Spectrophotometer (AAS) on flame mode with hollow cathode lamps of metal under analysis. The concentration of metal is displayed on the monitor. The standards of the metallic parameters were prepared from National Institute of Standards and Technology (NIST) certified (Certified Reference Materials) CRM as per NABL guidelines of 17,025:2017.

a – b Spatial distribution map of pH and EC. c – h Spatial distribution map of TDS, AK, TH, Ca 2+ , Mg 2+ and Na + . i – n : spatial distribution map of K + , \({\text{HCO}}_{3}^{ - }\) , \({\text{SO}}_{4}^{2 - }\) , Cl − , F − and \({\text{NO}}_{3}^{ - }\) . o – t Spatial distribution map of Cu, Mn, Ag, Zn, Fe and Ni
The quality assurance and quality control (QA/QC) procedure of the data has been considered during the study. Approximately half of the volume (500 ml) of samples were specially separated and checked in the laboratory to ensure QA/QC mechanisms. The accuracy of the chemical analysis has been validated by charge balance errors and samples < 5% error were considered.
The inverse distance weighted (IDW) interpolation technique used in this study is now-adays an effective tool for spatial interpolation of groundwater quality parameters leading to the generation of spatial distribution maps (Magesh et al. 2013 ; Kawo and Shankar 2018 ; Balamurugan et al. 2020b ; Sarfo and Shankar 2020 ). The weights were assigned to various parameters at each location based on distance and were calculated, taking into consideration the closest specified locations. The distribution of each groundwater quality parameter has been demarcated in different zones on spatial distribution map viz. acceptable/desirable and permissible limits according to BIS (2012, 2015) and WHO ( 2017 ) for drinking purpose. The statistical analysis and correlation matrix of the analysed groundwater quality parameters have been laid down as shown in Tables 1 and 2 , respectively.
The water quality index (WQI)
The WQI has been determined using the drinking water quality standard recommended by the World Health Organization (WHO 2017 ). The Water Quality Index has been calculated using the weighted arithmetic method, which was originally proposed by Horton ( 1965 ) and developed by Brown et al. ( 1972 ). The weighted arithmetic water quality index (WQI) is represented in the following way:
where n = number of variables or parameters, W i = unit weight for the i th parameter, Q i = quality rating (sub-index) of the i th water quality parameter.
The unit weight ( W i ) of the various water quality parameters are inversely proportional to the recommended standards for the corresponding parameters.
where, W i = unit weight for the i th parameter, S n = standard value for i th parameters, K = proportional constant,
The value of K has been considered ‘1′ here and is calculated using the mentioned equation below:
According to Brown et al. ( 1972 ), the value of quality rating or sub-index ( Q i ) is calculated using the equation as given below:
where V o = observed value of i th parameter at a given sampling site, V i = ideal value of i th parameter in pure water, S n = standard permissible value of i th parameter.
All the ideal values (V i ) are taken as zero for drinking water except pH and dissolved oxygen (Tripathy and Sahu 2005 ). In case of pH, the ideal value is 7.0 (for natural/pure water) while the permissible value is 8.5 (for polluted water). Similarly, for dissolved oxygen, the ideal value is 14.6 mg/L while the standard permissible value for drinking water is 5 mg/L. Therefore, the quality rating for pH and Dissolved Oxygen are calculated from the equations respectively as shown below:
where, V pH = observed value of pH, V do = observed value of dissolved oxygen.
If, Q i = 0 implies complete absence of contaminants while 0 < Q i < 100 implies that, the contaminants are within the prescribed standard. When Q i > 100 implies that, the contaminants are above the standards.
The classification of water quality, based on its water quality index (WQI) after Brown et al. ( 1972 ); Chatterjee and Raziuddin ( 2002 ) and Shankar and Kawo ( 2019 ) have been considered here in this study for further reference which is mentioned in Table 3 .
Result and discussion
Groundwater quality parameters.
In this study based on the selected parameters as discussed above the groundwater quality maps have been prepared with the help of ArcGIS software 10.1 as shown in Fig. 2 a–t. In the following lines, the various parameters considered in the study are being discussed: The Bureau of Indian Standard (BIS 2012, 2015) and World Health Organization (WHO 2017 ) of drinking water standards have been considered as a reference in this study.
Hydrogen ion concentration (pH)
It is an important indicator for assessing the quality and pollution of any aquifer system as it is closely related to other chemical constituents of water. The presence of hydrogen ion concentration is measured in terms of pH range. Water, in its pure form shows a neutral pH which indicates hydrogen ion concentration. In the present study, the range of pH varies between 6.81 (minimum) to 8.32 (maximum) which is within the acceptable limit (6.5–8.5, avg: 7.81) indicating the alkaline nature of groundwater (ideal range of pH for human consumption: 6.5–8.5).
Electrical conductivity (EC)
In fact, it is a measure of the ability of any substance or solution to conduct electrical current through the water. EC is directly proportional to the dissolved material in a water sample. The desirable limit of EC for drinking purpose is 750 µS/cm. In this study, the electrical conductivity varies between 286 and 1162 µS/cm. High EC at some sites suggests the mixing of sewage in groundwater as these sites are near dense urbanization.
Total dissolved solids (TDS)
The weight of residue expresses it after a water sample is evaporated to dry state. It includes calcium, magnesium, sodium, potassium, carbonate, bicarbonate, chloride and sulfate. In the present study, it ranges between 280 to 879 mg/l (< 500 mg/l TDS for potable water as per BIS.). The agricultural practices, residential runoff, leaching of soil causing contamination and point source water pollution discharge from industrial or sewage treatment plants are the primary sources for TDS (Boyd 2000 ).
Alkalinity (AK)
It is a measure of the carbonate, bicarbonate and hydroxide ions present in water. The desirable limit of alkalinity in potable water is 200 mg/l, above which the taste of water becomes unpleasant. In the study area, the alkalinity ranges between 50 to 452 mg/l, which is within the permissible limit (600 mg/l).
Total hardness (TH)
It is the amount of dissolved calcium and magnesium in the water. Water moving through soil and rock dissolves naturally occurring minerals and carries them into the groundwater as it is a great solvent for calcium and magnesium. In this study, hardness ranges between 70 to 592 mg/l, which is within the permissible limits (600 mg/l). The high concentration of TH in groundwater may cause heart disease and kidney stone in human beings.
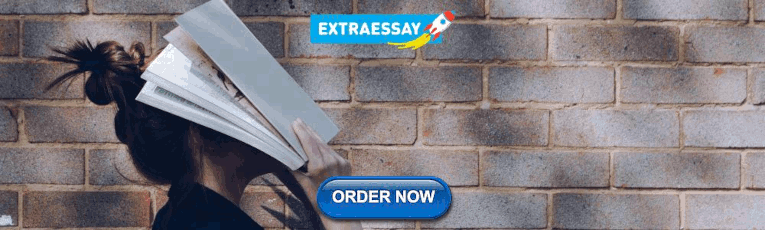
Calcium (Ca 2+ )
It enters into the aquifer system from the leaching of calcium bearing minerals. In the study area, the calcium concentration ranges from 12 to 112 mg/l and is within the permissible limit (200 mg/l). The lesser concentration of Ca 2+ in the groundwater satisfies the chemical weathering and dissolution of fluorite, consequently resulting in an increase of fluoride concentration.
Magnesium (Mg 2+ )
It is an important parameter responsible for the hardness of the water. In the study area, the concentration ranges between 2.4 to 120 mg/l and is present in little excess of the permissible limit (100 mg/l).
Sodium (Na + )
It is a highly reactive alkali metal. It is present in most of the groundwater. Many rocks and soils contain sodium compounds, which easily dissolves to liberate sodium in groundwater. In the study area, it ranges from 48.71 to 244.4 mg/l. The high concentration of Na + indicates weathering of rock-forming minerals i.e., silicate minerals (alkali feldspars) and/or dissolution of soil salts present therein due to evaporation (Stallard and Edmond 1983 ). In the aquifers, the high Na + concentration in groundwater may be related to the mechanism of cation exchange (Kangjoo Kim and Seong-Taekyun 2005).
Potassium (K + )
It is present in many minerals and most of the rocks. Many of these rocks are relatively soluble and releases potassium, the concentration of which increases with time in groundwater. In this study, it varies between 0.87 to 2.7 mg/l.
Bicarbonate ( \({\text{HCO}}_{3}^{ - }\) )
It is produced by the reaction of carbon dioxide with water on carbonate rocks viz. limestone and dolomite. The carbon-dioxide present in the soil reacts with the rock-forming minerals is responsible for the presence of bicarbonate, producing an alkaline environment in the groundwater. In the study area it varies between 36.61 to 536.95 mg/l and is within the permissible limit of 600 mg/l.
Sulfate ( \({\text{SO}}_{4}^{2 - }\) )
It is dissolved and leached from rocks containing gypsum, iron sulfides, and other sulfur bearing compounds. In the present study, it ranges between the 2.23 to 75.17 mg/l, which is well within the acceptable limit of 200 mg/l.
Chloride (Cl − )
In the present study the Cl − ranges between 70.92 to 276.59 mg/l which exceed the permissible limit (250 mg/l). The higher value of chlorine in groundwater makes it hazardous to human health (Pius et al. 2012 ; Sadat-Noori et al. 2014 ).
Fluoride (F − )
In groundwater fluoride is geogenic in nature. It is the lightest halogen, and one of the most reactive elements (Kaminsky et al. 1990 ). It usually occurs either in trace amounts or as a major ion with high concentration (Gaciri and Davies 1993 ; Apambire et al. 1997 ; Fantong et al. 2010 ). The groundwater contains fluorides released from various fluoride-bearing minerals mainly as a result of groundwater-host rock interaction. The study area comprising granite, granitic gneiss etc. is commonly found to contain fluorite (CaF 2 ) as an accessory mineral (Ozsvath 2006 ; Saxena and Ahmed 2003 ) which plays a significant role in controlling the geochemistry of fluoride (Deshmukh et al. 1995 ). In addition to fluorite it is also abundant in other rock-forming minerals like apatite, micas, amphiboles, and clay minerals (Karro and Uppin 2013 ; Narsimha and Sudarshan 2013 ; Naseem et al. 2010 ; Jha et al. 2010 ; Rafique et al. 2009 ; Carrillo-Rivera et al. 2002 ). In the present study, the fluoride concentration ranges from 0.11 to 3.91 mg/l. The concentration of fluoride exceeds the permissible limit (1.5 mg/l) in about 25% of the groundwater samples.
Nitrate ( \({\text{NO}}_{3}^{ - }\) )
Nitrate is naturally occurring ions and is a significant component in the nitrogen cycle. However, nitrate ion in groundwater is undesirable as it causes Methaemoglobinaemia in infants less than 6 months of age (Egereonu and Nwachukwu 2005 ). In general, its higher concentration causes health hazards if present beyond the permissible limit, 45 mg/l (Kumar et al. 2012 , 2014 ). In the study area, its concentration ranges from 86.95 to 210.4 mg/l. It is in excess of the permissible limits throughout the study area. The higher values of nitrate in potable water increases the chances of gastric ulcer/cancer, and other health hazards to infants and pregnant women (Rao 2006 ) also birth malformations and hypertension (Majumdar and Gupta 2000 ). The area under study is granite-gneiss terrain where the atmospheric nitrogen is fixed and added to the soil as ammonia through lightning storms, bacteria present in soil and root of plants. Further, animal wastes, plants and animals remain also undergo ammonification in the soil producing ammonia which undergoes nitrification/ammonia oxidation by Nitrosomonas and Nitrobacter bacteria to form nitrate (Rivett et al. 2008 ; Galloway et al. 2004). Granitic rocks contain nitrogen concentrations up to 250 mg Nkg −1 with ammonium partitioned into the orthoclase feldspar to a greater extent than muscovite or biotite (Boyd et al. 1993 ). Geologic nitrogen (nitrogen contained in bedrock) contribute to the ecosystem with nitrogen saturation (more nitrogen available than required by biota) leading to leaching of nitrogen and consequently elevating nitrate concentrations in groundwater (Dahlgren 1994 ; Holloway et al. 1998 ). Nitrogen released through weathering has a greater impact on soil and water quality. Also, denitrification is significant in modifying the level to which nitrogen released through weathering of bedrock influencing the supply of nitrate in groundwater (McCray et al. 2005 ).
Copper (Cu)
It is a naturally occurring metal in rock, soil, plants, animals, and groundwater in very less concentration. The concentration of Cu may get enriched into the groundwater through quarrying and mining activities, farming practices, manufacturing operations and municipal or industrial waste released. Cu gets into drinking water either by contaminating of well water or corrosion of copper pipes in case of water is acidic. In this study, it ranges between 0 and 0.0078 mg/l, which is within the permissible limit (0.05 mg/l).
Manganese (Mn)
It occurs naturally in groundwater, especially in an anaerobic environment. The concentrations of Mn in groundwater is dependent upon rainfall chemistry, aquifer lithology, geochemical environment, groundwater flow paths and residence time, etc. which may vary significantly in space and time. It may be released by the leaching of the overlying soils and minerals in underlying rocks as well as from the minerals of the aquifer itself in groundwater. In the present study, manganese ranges between 0.005 and 0.221 mg/l, which is within the permissible limit (0.3 mg/l).
It naturally occurs usually in the form of insoluble and immobile oxides, sulfides and some salts. It is rarely present in groundwater, surface water and drinking water at concentrations above 5 µg/litre (WHO 2017 ). In the present study, the silver ranges between 0.000 and 0.021 mg/l, which is within the permissible limit (0.1 mg/l).
Though it occurs in significant quantities in rocks, groundwater seldom contains zinc above 0.1 mg/l. In the present study, the groundwater shows the negligible concentration of Zn (0.0136 mg/l) which is well within the acceptable limit (5 mg/l).
The most common sources of iron in groundwater is weathering of iron-bearing minerals and rocks. The iron occurs naturally in the reduced Fe 2+ state in the aquifer, but its dissolution increases its concentration in groundwater. Iron in this state is soluble and generally does not create any health hazard. If Fe 2+ state is oxidised to Fe 3+ state in contact with atmospheric oxygen or by the action of iron-related bacteria which forms insoluble hydroxides in groundwater. So, the concentration of iron in groundwater is often higher than those measured in surface water. In the present study, the iron ranges between 0.0994 and 0.4018 mg/l, which is within of the permissible limit 1.0 mg/l (BIS 2015).
Nickel (Ni)
The primary source of nickel in groundwater is from the dissolution of nickel ore bearing rocks. The source of nickel in drinking water is leaching from metals in contact such as water supply pipes and fittings. Ni usually occurs in the divalent state, but oxidation states of + 1, + 3, or + 4 may also exist in nature. In the study area, it ranges between 0 and 0.0408 mg/l, and it crosses the permissible limit (0.02 mg/l).
Statistical analysis, correlation matrix and relative weightage
The relative weightage, general statistical analysis and correlation matrix of groundwater quality parameters are tabulated in Tables 4 , 1 and 2 , respectively. The correlation matrix of various 20 groundwater quality parameters, including 6 heavy metals was created and has been analysed using MS Excel 2016 Table 2 . Out of these, eight parameters viz. TDS, EC, Na + , Alkalinity, TH, Ca 2+ , Mg 2+ , \({\text{HCO}}_{3}^{ - }\) are significantly correlated, reflecting more than 0.50 correlation value. Further, TDS vs EC, Na + vs Alkalinity, TH as CaCO 3 − vs Ca 2+ and Mg 2+ , \({\text{HCO}}_{3}^{ - }\) vs Alkalinity and Na + indicates most relevant correlation having a significant impetus on the overall assessment of the quality of groundwater than any other major radicals and physical parameters. However, the majority of quality parameters are positively correlated with each other. A critical analysis of the correlation matrix for the heavy metals indicates that Cu is positively correlated with EC, TDS, Na + , K + , Cl − and \({\text{NO}}_{3}^{ - }\) . Similarly, Mn is positively correlated with pH, EC, TDS and Cu. While, Ag is positively correlated with pH, Ca 2+ , Mg 2+ , K + , TH, Cl − , \({\text{NO}}_{3}^{ - }\) and Mn. Further, Fe is positively correlated with TDS, Mg 2+ , Na + , TH, \({\text{HCO}}_{3}^{ - }\) , \({\text{SO}}_{4}^{2 - }\) , \({\text{NO}}_{3}^{ - }\) , Cu and Ag. Similarly, Ni is positively correlated with pH, EC, TDS, Ca 2+ , K + , \({\text{NO}}_{3}^{ - }\) and Mn.
The higher concentration of Ni, Fe and Mn may trigger the presence of other heavy metals viz. Pb, Cd and Cr which are very sensitive and significant heavy metal and needs to be observed carefully in future for groundwater quality in the study area. The presence of Fe, \({\text{SO}}_{4}^{2 - }\) and \({\text{NO}}_{3}^{ - }\) may trigger the presence of Cd (Chaurasia et al. 2018 ).
Spatial distribution pattern
The spatial distribution pattern of the contour maps of the groundwater quality parameters have been generated as represented in Fig. 2 a–t. The spatial distribution pattern of the pH indicates that the central part along NW–SE across the district with some scattered small patches throughout indicating the presence of alkaline groundwater (Fig. 2 a). In acidic water, fluoride is adsorbed on a clay surface, while in alkaline water, fluoride is desorbed from solid phases; therefore, alkaline pH is more favourable for fluoride dissolution, (Keshavarzi et al. 2010 ; Rafique et al. 2009 ; Saxena and Ahmed 2003 ; Rao 2009 ; Ravindra and Garg 2007 ; Vikas et al. 2009 ). The southern portion of the district in Kabrai Block is having high TDS (> 750 mg/l) in groundwater (Fig. 2 c) due to poor fluxing and highly weathered rock formations. Similarly, EC is mainly highest (> 900 mg/l) in the southern part with small scattered patches in central and NE part of the district (Fig. 2 b). This is in consonance with the higher TDS (significant positive correlation with EC) as evidenced by the correlation matrix of the quality parameters (Table 2 ). The alkalinity map clearly and significantly indicates that it is highest in the central part surrounded by gradually decreasing alkalinity outwards (Fig. 2 d). The bicarbonates trigger the alkalinity in groundwater (Adams et al. 2001 ). The quality of groundwater in a major portion of the study area is alkaline in nature, indicating that the dissolved carbonates are predominantly in the form of bicarbonates. A positive correlation is observed between the alkalinity of groundwater and fluoride (Table 2 ), consequently releasing fluoride in the groundwater. The spatial distribution map of Ca 2+ suggests varying concentration within permissible limit throughout the study area (Fig. 2 f) due to the presence of alkali feldspar in granite. Similarly, Mg 2+ is also distributed unevenly but falls within permissible limit with an exception in NE part of the district (Fig. 2 g). The spatial distribution pattern of TH reflects that the study area is characterized by moderately hard groundwater.
Figure 2 e The Ca 2+ and Mg 2+ ions present in the groundwater are possibly derived from leaching of calcium and magnesium bearing rock-formations in the study area. The fluoride in groundwater shows a negative correlation with Ca 2+ , indicating the high value of fluoride in groundwater in association with low Ca 2+ content. The correlation matrix clearly marks a significant positive correlation among Na + , alkalinity and TDS, which is being reflected from their respective spatial distribution maps (Fig. 2 c, d and h). Na + is highest in the central part (with small patches in the eastern part and insignificantly in the western part) which is in conformity with the alkalinity and TDS spatial distribution patterns. Although, the presence of K + is insignificant and its lower concentration within the permissible limit is covering a major portion of the district due to poor weathering of orthoclase. Its distribution pattern indicates conformity more or less with the TDS and Na + (Fig. 2 c, h, and i). \({\text{HCO}}_{3}^{ - }\) is an important quality parameter showing significant positive correlation (> 0.50) with alkalinity and Na + (Table 2 ) which is also reflected in the spatial distribution pattern of these parameters (Fig. 2 d, h, j). Although sulphate ( \({\text{SO}}_{4}^{2 - }\) ) is an important quality parameter. It is present within the permissible limit in the study.
area (Fig. 2 k). Chloride is slightly in excess in a larger patch, particularly in SE-part of the study area which may cause a health hazard. It is revealed from the spatial distribution map of chloride (Fig. 2 l). This is due to poor fluxing and presence of halite mineral. Fluoride (F − ) is an important quality parameter, especially with respect to the study area where it is present noticeably in scattered patches throughout the district. It is observed that mainly in NE part, the central part and SE part of the district the concentration of fluoride is in excess (2.82 mg/l to 3.91 mg/l) of permissible limit 1.5 mg/l (Fig. 2 m). The higher concentration (> 3.0 mg/l) of fluoride may lead to skeletal fluorosis (Raju et al 2009 ). Several factors viz. temperature, pH, presence or absence of complexing or precipitating ions and colloids, the solubility of fluorine bearing minerals (biotite and apatite), anion exchange capacity of the aquifer (OH − with F − ), size and type of geological formations traversed by groundwater and the contact time during which water remains in contact with the formation are responsible for fluoride concentration in groundwater (Apambire et al. 1997 ). The lithology of fractured rock reveals that it contains more fluoride bearing minerals than massive rocks (Pandey et al. 2016 ). Nitrate (NO 3 − ) in groundwater is mainly anthropogenic in nature which could be due to leaching from waste disposal, sanitary landfills, over-application of inorganic nitrate fertilizer or improper manure management practice (Chapman 1996 ). In this study, it is observed that nitrate is in excess of the permissible limits with varying degree of concentration throughout the district, causing health hazard (Fig. 2 n). The area under study is granite-gneiss terrain where the atmospheric nitrogen is fixed and added to the soil as ammonia through lightning storms, bacteria present in soil and plants roots. Further, animal wastes, plants and animals remain also undergo ammonification in the soil producing ammonia which undergoes nitrification. The high values of nitrate in groundwater samples in the area may be due to unlined septic tanks and unplanned sewerage system that contaminates to the phreatic aquifer (Hei et al. 2020 ). Proper monitoring and concerned regulated effort are consistently required to get the assessment of nitrate impact on human health.
As far as heavy metals concentration in groundwater is concerned, Cu does not mark its noticeable presence (Fig. 2 o). Another, naturally occurring quality parameter is Mn which shows its presence within the permissible limit (Fig. 2 p). Silver and Zinc do not show any remarkable presence in the study area (Fig. 2 q and r). The study reveals a higher concentration of iron in groundwater in the Eastern part of the district due to secondary porosity and where ferrous (Fe 2+ ) ion usually occurs below the water table. The Fe 2+ after converting into Ferric (Fe 3+ ) state, becomes harmful and precipitated. This condition can be avoided naturally by raising the water table through groundwater recharging the affected area (Fig. 2 s). Nickel shows its remarkable presence in smaller patches in different areas (Fig. 2 t) due to the presence of heavy minerals like rutile and apatite.
Water quality index
The water quality index (WQI) map has been prepared using ArcGIS 10.1 on the basis of the selectively chosen quality parameters to decipher the various quality classes viz. excellent, good, poor, very poor and unsuitable at each hydro-station for drinking purpose (Tables 3 and 5 ; Fig. 3 ). The WQI Map of the study area indicates that major portion is having excellent (0–25) quality of groundwater while very poor (75–100) to unsuitable (> 100) quality is prevailing in small pockets in SW part (Fig. 3 ). The map clearly indicates that the quality of groundwater in Panwari Block belongs to excellent to good categories as for as potability for human consumption is concerned.
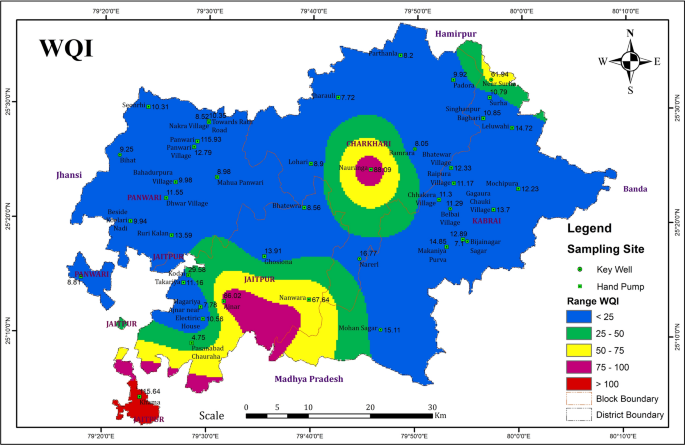
Water quality index map of the study area, District Mahoba
There is gradual variation in groundwater quality from very poor to excellent at the central part and outwards in the Charkhari Block. There is no noticeable change in the quality of groundwater except in the SW part of the Kabari Block. In the Jaitpur block, there is a significant.
variation in the quality class and the SW part (Nanwara, Ajnar and Khama) is characterized by poor, very poor and unsuitable categories (Fig. 3 ). Remaining part of the block falls under good to excellent groundwater quality. Overall, the quality of groundwater belongs to the excellent category in a major portion of the study area and is suitable for drinking as well as domestic uses.
Hydro-chemical facies
The major ions analysed are unevenly distributed and have been plotted on a Hill-Piper Trilinear diagram (Fig. 4 ). This diagram is comprised of two triangles at the base and one diamond shape at the top to represent the major significant cations and anions responsible for the nature of groundwater (Balamurugan et al. 2020a ). The piper diagram is used to categorize groundwater into various six types such as Ca 2+ - \({\text{HCO}}_{3}^{ - }\) type, Na + -Cl − type, mixed Ca 2+ -Mg 2+ -Cl − type, Ca 2+ -Na + - \({\text{HCO}}_{3}^{ - }\) type, Na + - \({\text{HCO}}_{3}^{ - }\) type and Ca 2+ -Cl − type. A critical evaluation of the diagram reflects that 32.56% of the samples fall under Na + -Cl − type, 30.23% of the samples under mixed Ca 2+ -Mg 2+ -Cl − type, 16.28% of the samples under Ca 2+ - \({\text{HCO}}_{3}^{ - }\) type, 13.95% of the samples under mixed Ca 2+ -Na + - \({\text{HCO}}_{3}^{ - }\) type, 4.65% of the samples under Na + - \({\text{HCO}}_{3}^{ - }\) type and 2.33% of the samples under Ca 2+ -Cl − type. Further, the observation reveals that the samples are distributed mainly into Na + -Cl − type, mixed Ca 2+ -Mg 2+ -Cl − type and Ca 2+ - \({\text{HCO}}_{3}^{ - }\) type reflecting higher concentration of sodium and calcium bearing salt/mineral. Hydrochemistry of the analysed samples indicate that the major cations are present in order Na + > Ca 2+ > Mg 2+ > K + of mean abundance while anions are present in the mean abundance order of \({\text{HCO}}_{3}^{ - }\) > \({\text{NO}}_{3}^{ - }\) > Cl − > \({\text{SO}}_{4}^{2 - }\) > F − (Table 1 ). This reveals that sodium, chloride and bicarbonate dominate the ionic concentration in the groundwater due to action of weathering of minerals like halite and dolomite as well as ion exchange process.
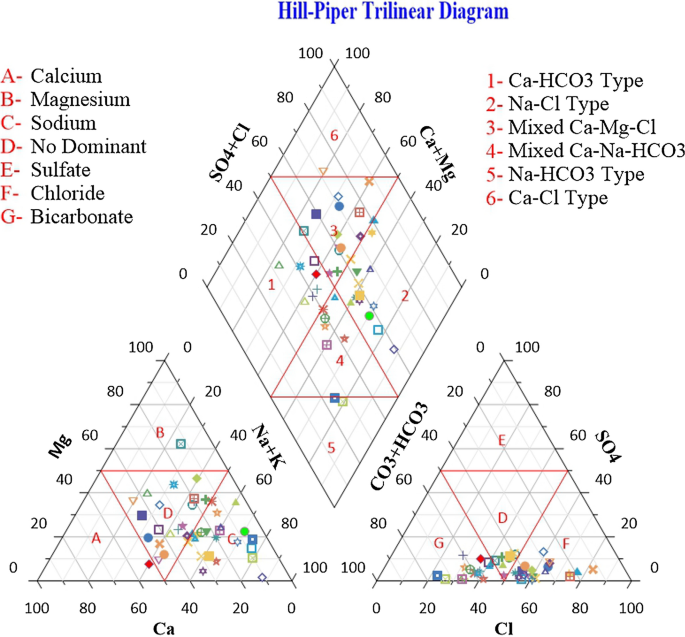
Types of groundwater
The outcome of the present research in the hard rock area of the Bundelkhand region of India reveals that the groundwater has been deteriorated due to both geogenic and anthropogenic activities.
The study area is comprised mainly of granite and alkali granite, specifically in extreme southern which is responsible for leaching of fluoride in groundwater.
The thickness of overburden (loose soil and weathered rock) in the northern part of the study area is negligible. Therefore, there is a poor fluxing of groundwater which in turn triggers the concentration of TDS, fluoride and bicarbonate in groundwater.
Anthropogenic activities like unlined septic tanks and unplanned sewerage system have triggered the nitrate concentration in groundwater, particularly in the central and northern part of the study area. The rest of the area is safe and has potable groundwater. In addition, the area under study is granite-gneiss terrain where the atmospheric nitrogen is fixed and added to the soil as ammonia through natural lightning, bacteria present in soil and plants roots. Further, ammonification of animal wastes, plants and animal remains produces ammonia which undergoes nitrification.
Hydro-chemical facies reveal that the nature of groundwater is Na + -Cl − , mixed Ca 2+ -Mg 2+ -Cl − and Ca 2+ - \({\text{HCO}}_{3}^{ - }\) type in the study area.
The high value of WQI has been found, which is due to the higher values of chloride, fluoride, nitrate, manganese, iron, and nickel in the groundwater, which warrants immediate attention.
On the basis of WOI, it is concluded that the groundwater is safe and potable in the study area except for localized pockets in Jaitpur and Charkhari Blocks.
Adams S, Titus R, Pietersen K, Tredoux G, Harris C (2001) Hydrochemical characteristics of aquifers near Sutherland in the Western Karoo, South Africa. J Hydrol 241:91–103
Article Google Scholar
Aghazadeh N, Chitsazan M, Golestan Y (2017) Hydrochemistry and quality assessment of groundwater in the Ardabil area. Iran Appl Water Sci 7:3599–3616
Alobaidy AM, Abid HS, Maulood BK (2010) Application of water quality index for assessment of Dokan lake ecosystem, Kurdistan region, Iraqi. J Water Res Prot 2:792–798
Apambire WB, Boyle DR, Michel FA (1997) Geochemistry, genesis, and health implications of fluoriferous groundwater in the upper regions of Ghana. Environ Geol 33:13–22
APHA, AWWA, WEF (2017) Standard Methods for the Examination of Water and Wastewater. 23th edition., APHA, Washington, DC. v.6.
Aravindan S, Shankar K, Ganesh BP, Rajan KD (2010) Hydrogeochemical mapping of in the hard rock area of Gadilam River basin, using GIS technique. Tamil Nadu Indian J Appl Geochem 12(2):209–216
Google Scholar
Balamurugan P, Kumar PS, Shankar K (2020a) Dataset on the suitability of groundwater for drinking and irrigation purposes in the Sarabanga River region, Tamil Nadu, India. Data in Brief 29:105255
Balamurugan P, Kumar PS, Shankar K, Nagavinothini R, Vijayasurya K (2020b) Non-carcinogenic risk assessment of groundwater in Southern Part of Salem District in Tamilnadu, India. J Chil Chem Soc 65:4697–4707
Bawoke GT, Anteneh ZL (2020) Spatial assessment and appraisal of groundwater suitability for drinking consumption in Andasa watershed using water quality index (WQI) and GIS techniques: Blue Nile Basin. Northwestern Ethiopia Cogent Eng 7(1):1748950
BIS (Bureau of Indian Standard) (2012) Indian standard drinking water specification, second revision, pp.1–16.
BIS (Bureau of Indian Standard) (2015) Indian standard drinking water specification. second revision, pp.2–6.
Boyd CE (2000) Water Quality an Introduction. Kluwer Acadamic Publi-shers, Boston, USA, p 330
Boyd SR, Hall A, Pillinger CT (1993) The measurement of d15N in crustal rocks by static vacuum mass spectrometry: Application to the origin of the ammonium in the Cornubian batholith, southwest England. Geochim Cosmochim Acta 57:1339–1347
Brhane GK (2018) Characterization of hydro chemistry and groundwater quality evaluation for drinking purpose in Adigrat area, Tigray, northern Ethiopia. Water Sci 32:213–229
Brown RM, Mccleiland NJ, Deiniger RA, O’Connor MF (1972) Water quality index-crossing the physical barrier. Res Jerusalem 6:787–797
Carrillo-Rivera JJ, Cardona A, Edmunds WM (2002) Use of abstraction regime and knowledge of hydrogeological conditions to control high-fluoride concentration in abstracted groundwater: San Luis Potosı basin, Mexico. J Hydrol 261:24–47
Cerar S, Urbanc J (2013) Carbonate chemistry and isotope characteristics of groundwater of Ljubljansko polje and Ljubljansko Barje aquifers in Slovenia. Sci World J 2013:1–11
Chapman D (1996) Water Quality Assessment-A guide to use of biota, sediments and water environmental monitoring 2nd Edition EPFN Spon. London. p.626.
Chatterjee C, Raziuddin M (2002) Determination of Water Quality Index (WQI) of a degraded river in Asansol industrial area (West Bengal). Nat Environ Pollut Technol 1:181–189
Chaurasia AK, Pandey HK, Tiwari SK, Prakash R, Pandey P, Ram A (2018) Groundwater quality assessment using water quality index (WQI) in parts of Varanasi district, Uttar Pradesh, India. J Geol Soc India 92:76–82
Dahlgren RA (1994) Soil acidification and nitrogen saturation from weathering of ammonium-bearing rock. Nature 368:838–841
Deshmukh AN, Shah KC, Sriram A (1995) Coal Ash: a source of fluoride pollution, a case study of Koradi thermal power station, District Nagpur. Maharashtra Gondwana Geol Mag 9:21–29
Diersing N, Nancy F (2009) Water quality: Frequently asked questions. Florida Brooks National Marine Sanctuary, Key West, FL
Egereonu UU, Nwachukwu UL (2005) Evaluation of the surface and groundwater resources of Efuru River Catchment, Mbano, South Eastern. Nigeria J Assoc Adv Model Simulat Tech Enterpr 66:53–71
Fantong WY, Satake H, Ayonghe SN, Suh EC, Adelana SM, Fantong EBS, Zhang J (2010) Geochemical provenance and spatial distribution of fluoride in groundwater of Mayo Tsanaga River Basin, Far North Region, Cameroon: implications for incidence of fluorosis and optimal consumption dose. Environ Geochem Health 32:147–163
Gaciri SJ, Davies TC (1993) The occurrence and geochemistry of fluoride in some natural waters of Kenya. J Hydrol 143:395–412
Galloway JN, Dentener FJ, Capone DG, Boyer EW, Howarth RW, Seitzinger SP, Asner GP, Cleveland CC, Green PA, Holland EA, Karl DM (2004) Nitrogen cycles: past, present, and future. Biogeochemistry 70:153–226
Gebrehiwot AB, Tadesse N, Jigar E (2011) Application of water quality index to assess suitablity of groundwater quality for drinking purposes in Hantebet watershed, Tigray, Northern Ethiopia. ISABB J Food Agric Sci 1:22–30
GEMS U (2007) Global drinking water quality index development and sensitivity analysis report. United Nations Environment Programme Global Environment Monitoring System/Water Programme. p.58.
Hei L, Bouchaou L, Tadoumant S, Reichert B (2020) Index-based groundwater vulnerability and water quality assessment in the arid region of Tata city (Morocco). Groundw Sustain Dev:100344
Holloway JM, Dahlgren RA, Hansen B, Casey WH (1998) Contribution of bedrock nitrogen to high nitrate concentrations in stream water. Nature 395:785–788
Horton RK (1965) An index number system for rating water quality. J Water Pollut Control Federation 373:303–306
Jagadeeswari PB, Ramesh K (2012) Deciphering fresh and saline groundwater interface in south Chennai coastal aquifer, Tamil Nadu, India. Int J Res Chem Environ 2:123–132
Jha SK, Nayak AK, Sharma YK (2010) Potential fluoride contamination in the drinking water of Marks Nagar, Unnao district, UP, India. Environ Geochem Health 32:217–226
Johnson DL, Ambrose SH, Bassett TJ, Bowen ML, Crummey DE, Isaacson JS, Winter-Nelson AE (1997) Meanings of environmental terms. J Environ Qual 26:581–589
Kalavathy S, Sharma TR, Suresh KP (2011) Water quality index of river Cauvery in Tiruchirappalli district, Tamilnadu. Arch Environ Sci 5:55–61
Kaminsky LS, Mahoney MC, Leach J, Melius J, Jo Miller M (1990) Fluoride: benefits and risks of exposure. Crit Rev Oral Biol Med 1:261–281
Kangjoo K, Yun ST (2005) Buffering of sodium concentration by cation exchange in the groundwater system of a sandy aquifer. Geochem J 39:273–284
Karro E, Uppin M (2013) The occurrence and hydrochemistry of fluoride and boron in carbonate aquifer system, central and western Estonia. Environ Monit Assess 185:3735–3748
Kavitha MT, Divahar R, Meenambal T, Shankar K, VijaySingh R, Haile TD, Gadafa C (2019a) Dataset on the assessment of water quality of surface water in Kalingarayan Canal for heavy metal pollution, Tamil Nadu. Data in Brief 22:878–884
Kavitha MT, Shankar K, Divahar R, Meenambal T, Saravanan R (2019b) Impact of industrial wastewater disposal on surface water bodies in Kalingarayan canal, Erode district, Tamil Nadu, India. Arch Agric Environ Sci 4(4):379–387
Kavitha R, Elangovan K (2010) Ground water quality characteristics at Erode district, Tamilnadu India. Int J Environ Sci 1:163–175
Kawo NS, Shankar K (2018) Groundwater quality assessment using water quality index and GIS technique in Modjo River Basin, Central Ethiopia. J Afr Earth Sci 147:300–311
Keshavarzi B, Moore F, Esmaeili A, Rastmanesh F (2010) The source of fluoride toxicity in Muteh area, Isfahan. Iran Environ Earth Sci 61:777–786
Khan D, Hagras MA, Iqbal N (2014) Groundwater quality evaluation in Thal Doab of Indus Basin of Pakistan. Int J Modern Eng Res 4:36–47
Kumar SK, Chandrasekar N, Seralathan P, Godson PS, Magesh NS (2012) Hydrogeochemical study of shallow carbonate aquifers, Rameswaram Island, India. Environ Monit Assess 184:4127–4138
Kumar SK, Logeshkumaran A, Magesh NS, Godson PS, Chandrasekar N (2014) Hydro-geochemistry and application of water quality index (WQI) for groundwater quality assessment, Anna Nagar, part of Chennai City, Tamil Nadu, India. Appl Water Sci 5:335–343
Magesh NS, Elango L (2019) Spatio-temporal variations of fluoride in the groundwater of Dindigul District, Tamil Nadu, India: a comparative assessment using two interpolation techniques. GIS Geostat Techn Groundwater Sci:283–296.
Magesh NS, Krishnakumar S, Chandrasekar N, Soundranayagam JP (2013) Groundwater quality assessment using WQI and GIS techniques, Dindigul district, Tamil Nadu, India. Arab J Geosci 6:4179–4189
Majumdar D, Gupta N (2000) Nitrate pollution of groundwater and associated human health disorders. Indian J Environ Health 42:28–39
McCray JE, Kirkland SL, Siegrist RL, Thyne GD (2005) Model parameters for simulating fate and transport of on-site wastewater nutrients. Ground Water 43:628–639
Modibo Sidibé A, Lin X, Koné S (2019) Assessing groundwater mineralization process, quality, and isotopic recharge origin in the Sahel Region in Africa. Water 11:789
Mostafa MG, Uddin SMH, Haque ABMH (2017) Assessment of hydrogeochemistry and groundwater quality of Rajshahi City in Bangladesh. Appl Water Sci. 7:4663–4671
Narsimha A, Sudarshan V (2013) Hydrogeochemistry of groundwater in Basara area, Adilabad District, Andhra Pradesh, India. J Appl Geochem 15:224–237
Naseem S, Rafique T, Bashir E, Bhanger MI, Laghari A, Usmani TH (2010) Lithological influences on occurrence of high-fluoride groundwater in Nagar Parkar area, Thar Desert, Pakistan. Chemosphere 78:1313–1321
Ozsvath DL (2006) Fluoride concentrations in a crystalline bedrock aquifer Marathon County, Wisconsin. Environ Geol 50:132–138
Panda RB, Sahu BK, Garnaik BK, Sinha BK, Nayak A (1991) Investigation of water quality of Brahmani River. Indian J Environ Health 33:45–50
Pandey HK, Duggal SK, Jamatia A (2016) Fluoride contamination of groundwater and it’s hydrogeological evolution in District Sonbhadra (UP) India. Proc Natl Acad Sci, India, Sect A 86:81–93
Panigrahi T, Das KK, Dey BS, Panda RB (2012) Assessment of Water Quality of river Sono, Balasore. Int J Environ Sci 3:49–56
Panneerselvam B, Paramasivam SK, Karuppannan S et al (2020a) A GIS-based evaluation of hydrochemical characterisation of groundwater in hard rock region, South Tamil Nadu. India Arab J Geosci 13(17):1–22
Panneerselvam, B., Karuppannan, S., & Muniraj, K. (2020b). Evaluation of drinking and irrigation suitability of groundwater with special emphasizing the health risk posed by nitrate contamination using nitrate pollution index (NPI) and human health risk assessment (HHRA). Human and Ecological Risk Assessment: An Int J, pp 1–25.
Pius A, Jerome C, Sharma N (2012) Evaluation of groundwater quality in and around Peenya industrial area of Bangalore, South India using GIS techniques. Environ Monit Assess 184:4067–4077
Rafique T, Naseem S, Usmani TH, Bashir E, Khan FA, Bhanger MI (2009) Geochemical factors controlling the occurrence of high fluoride groundwater in the Nagar Parkar area, Sindh, Pakistan. J Hazard Mater 171:424–430
Raju NJ, Dey S, Das K (2009) Fluoride contamination in groundwater of Sonbhadra district, Uttar Pradesh, India. Curr Sci 96:979–985
Rao NS (2006) Nitrate pollution and its distribution in the groundwater of Srikakulam district, Andhra Pradesh, India. Environ Geol 51:631–645
Rao NS (2009) Fluoride in groundwater, Varaha River Basin, Visakhapatnam District, Andhra Pradesh, India. Environ Monit Assess 152:47–60
Ravindra K, Garg VK (2007) Hydro-chemical survey of groundwater of Hisar city and assessment of defluoridation methods used in India. Environ Monit Assess 132:33–43
Rivett MO, Russ SR, Morgan P, Smith JW, Bemment N, CD, (2008) Nitrate attenuation in groundwater: a review of biogeochemical controlling processes. Water Res 42:4215–4232
Sadat-Noori SM, Ebrahimi K, Liaghat AM (2014) Groundwater quality assessment using the Water Quality Index and GIS in Saveh-Nobaran aquifer. Iran Environ Earth Sci 71:3827–3843
Sarfo AK, Shankar K (2020) Application of geospatial technologies in the COVID-19 fight of Ghana. Transact Indian Nat Acad Eng 5:193–204
Saxena V, Ahmed S (2003) Inferring the chemical parameters for the dissolution of fluoride in groundwater. Environ Geol 43:731–736
Selvam S, Manimaran G, Sivasubramanian P (2013) Hydrochemical characteristics and GIS-based assessment of groundwater quality in the coastal aquifers of Tuticorin Corporation, Tamilnadu, India. Appl Water Sci 3:145–159
Shankar K, Aravindan S, Rajendran S (2010) GIS based groundwater quality mapping in Paravanar River Sub-Basin, Tamil Nadu, India. Int J Geomat Geosci 1:282–296
Shankar K, Aravindan S, Rajendran S (2011) Hydrogeochemistry of the Paravanar river sub-basin, Cuddalore District, Tamilnadu, India. E-J Chem 8:835–845
Shankar K, Aravindan S, Rajendran S (2011) Spatial distribution of groundwater quality in Paravanar river sub basin, Cuddalore district, Tamil Nadu. Int J Geomat Geosci 1:914–931
Shankar K, Kawo NS (2019) Groundwater quality assessment using geospatial techniques and WQI in North East of Adama Town, Oromia Region. Ethiopia Hydrospatial Anal 3(1):22–36
Soujanya Kamble B, Saxena PR, Kurakalva RM, Shankar K (2020) Evaluation of seasonal and temporal variations of groundwater quality around Jawaharnagar municipal solid waste dumpsite of Hyderabad city. India SN Appl Sci 2:498
Stallard RF, Edmond JM (1983) Geochemistry of the Amazon: 2. The influence of geology and weathering environment on the dissolved load. J Geophys Res: Oceans 88:9671–9688
Tripathy JK, Sahu KC (2005) Seasonal hydrochemistry of groundwater in the Barrier Spit system of the Chilika Lagoon, India. J Environ Hydrol 13:1–9
Venkateswaran S, Karuppannan S, Shankar K (2012) Groundwater quality in Pambar sub-basin, Tamil Nadu, India using GIS. Int J Recent Sci Res 3:782–787
Vikas C, Kushwaha RK, Pandit MK (2009) Hydrochemical status of groundwater in district Ajmer (NW India) with reference to fluoride distribution. J Geol Soc India 73:773–784
WHO (2017) Guideline for drinking water quality, 4th edn. World Health Organization, Geneva
Yisa J, Jimoh T (2010) Analytical studies on water quality index of river Landzu. Am J Appl Sci. 7:453–458
Download references
Acknowledgements
Our thanks go to Central Groundwater Board (CGWB), Lucknow, NR, Region and Department of Soil Science & Agricultural Chemistry, Banaras Hindu University, Varanasi for their valuable support during the chemical analysis of groundwater samples.
There is no sponsored/finical assistance is involved in the present research work.
Author information
Authors and affiliations.
Department of Geology, Institute of Science, CAS, Banaras Hindu University, Varanasi, 221005, India
Arjun Ram & Abhishek Kumar Chaurasia
UGC-HRDC, Banaras Hindu University, Varanasi, 221005, India
S. K. Tiwari
Department of Civil Engineering, Motilal Nehru National Institute of Technology Allahabad, Prayagraj, 211004, UP, India
H. K. Pandey
Central Groundwater Board, Lucknow, 226021, UP, India
Supriya Singh
Department of Soil Science & Agricultural Chemistry, Banaras Hindu University, Varanasi, 221005, India
Y. V. Singh
You can also search for this author in PubMed Google Scholar
Corresponding author
Correspondence to H. K. Pandey .
Ethics declarations
Conflict of interest.
This is certified that there is no conflict of interest either academic or commercial.
Ethical standards
The research work is original in nature and does not contain the others findings accept referred work. Therefore, the entire manuscript follows the Ethical Standards.
Additional information
Publisher's note.
Springer Nature remains neutral with regard to jurisdictional claims in published maps and institutional affiliations.
Rights and permissions
Open Access This article is licensed under a Creative Commons Attribution 4.0 International License, which permits use, sharing, adaptation, distribution and reproduction in any medium or format, as long as you give appropriate credit to the original author(s) and the source, provide a link to the Creative Commons licence, and indicate if changes were made. The images or other third party material in this article are included in the article's Creative Commons licence, unless indicated otherwise in a credit line to the material. If material is not included in the article's Creative Commons licence and your intended use is not permitted by statutory regulation or exceeds the permitted use, you will need to obtain permission directly from the copyright holder. To view a copy of this licence, visit http://creativecommons.org/licenses/by/4.0/ .
Reprints and permissions
About this article
Ram, A., Tiwari, S.K., Pandey, H.K. et al. Groundwater quality assessment using water quality index (WQI) under GIS framework. Appl Water Sci 11 , 46 (2021). https://doi.org/10.1007/s13201-021-01376-7
Download citation
Received : 27 May 2020
Accepted : 25 January 2021
Published : 12 February 2021
DOI : https://doi.org/10.1007/s13201-021-01376-7
Share this article
Anyone you share the following link with will be able to read this content:
Sorry, a shareable link is not currently available for this article.
Provided by the Springer Nature SharedIt content-sharing initiative
- Groundwater
- Hydrochemistry
- Piper diagram
- Bundelkhand massif
- Find a journal
- Publish with us
- Track your research
Thank you for visiting nature.com. You are using a browser version with limited support for CSS. To obtain the best experience, we recommend you use a more up to date browser (or turn off compatibility mode in Internet Explorer). In the meantime, to ensure continued support, we are displaying the site without styles and JavaScript.
- View all journals
- Explore content
- About the journal
- Publish with us
- Sign up for alerts
- Published: 22 April 2024
Global peak water limit of future groundwater withdrawals
- Hassan Niazi ORCID: orcid.org/0000-0001-6556-2854 1 ,
- Thomas B. Wild 1 ,
- Sean W. D. Turner ORCID: orcid.org/0000-0003-4400-9800 2 ,
- Neal T. Graham ORCID: orcid.org/0000-0001-6867-5475 1 ,
- Mohamad Hejazi ORCID: orcid.org/0000-0003-4194-2208 3 ,
- Siwa Msangi ORCID: orcid.org/0000-0002-5408-6909 4 ,
- Son Kim ORCID: orcid.org/0000-0001-5195-489X 1 ,
- Jonathan R. Lamontagne ORCID: orcid.org/0000-0003-3976-1678 5 &
- Mengqi Zhao ORCID: orcid.org/0000-0001-5385-2758 6
Nature Sustainability volume 7 , pages 413–422 ( 2024 ) Cite this article
477 Accesses
130 Altmetric
Metrics details
- Socioeconomic scenarios
- Sustainability
- Water resources
Over the past 50 years, humans have extracted the Earth’s groundwater stocks at a steep rate, largely to fuel global agro-economic development. Given society’s growing reliance on groundwater, we explore ‘peak water limits’ to investigate whether, when and where humanity might reach peak groundwater extraction. Using an integrated global model of the coupled human–Earth system, we simulate groundwater withdrawals across 235 water basins under 900 future scenarios of global change over the twenty-first century. Here we find that global non-renewable groundwater withdrawals exhibit a distinct peak-and-decline signature, comparable to historical observations of other depletable resources (for example, minerals), in nearly all (98%) scenarios, peaking on average at 625 km 3 yr −1 around mid-century, followed by a decline through 2100. The peak and decline occur in about one-third (82) of basins, including 21 that may have already peaked, exposing about half (44%) of the global population to groundwater stress. Most of these basins are in countries with the highest current extraction rates, including the United States, Mexico, Pakistan, India, China, Saudi Arabia and Iran. These groundwater-dependent basins will probably face increasing costs of groundwater and food production, suggesting important implications for global agricultural trade and a diminished role for groundwater in meeting global water demands during the twenty-first century.
This is a preview of subscription content, access via your institution
Access options
Access Nature and 54 other Nature Portfolio journals
Get Nature+, our best-value online-access subscription
24,99 € / 30 days
cancel any time
Subscribe to this journal
Receive 12 digital issues and online access to articles
111,21 € per year
only 9,27 € per issue
Buy this article
- Purchase on Springer Link
- Instant access to full article PDF
Prices may be subject to local taxes which are calculated during checkout
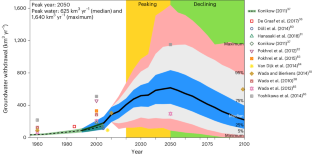
Similar content being viewed by others
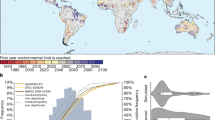
Environmental flow limits to global groundwater pumping
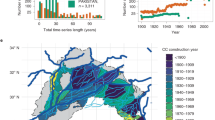
A century of groundwater accumulation in Pakistan and northwest India
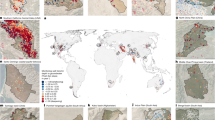
Rapid groundwater decline and some cases of recovery in aquifers globally
Data availability.
The large-ensemble data repository contains relevant model outputs from 900 scenarios simulated using GCAM 59 . The data repository is to be used in combination with the meta-repository containing scripts and files for reproducing the experiment as well as for the analysis and post-processing of the model outputs 60 .
Code availability
The meta-repository containing and explaining usage of code, modelling files and input data could be accessed to reproduce experiments, results and visualizations presented in this study 60 .
Abbott, B. W. et al. Human domination of the global water cycle absent from depictions and perceptions. Nat. Geosci. 12 , 533–540 (2019).
Article CAS Google Scholar
Qin, Y. et al. Flexibility and intensity of global water use. Nat. Sustain. 2 , 515–523 (2019).
Article Google Scholar
Postel Sandra, L., Daily Gretchen, C. & Ehrlich Paul, R. Human appropriation of renewable fresh water. Science 271 , 785–788 (1996).
Vörösmarty Charles, J., Green, P., Salisbury, J. & Lammers Richard, B. Global water resources: vulnerability from climate change and population growth. Science 289 , 284–288 (2000).
Oki, T. & Kanae, S. Global hydrological cycles and world water resources. Science 313 , 1068–1072 (2006).
Gleick, P. H. Transitions to freshwater sustainability. Proc. Natl Acad. Sci. USA 115 , 8863–8871 (2018).
Liu, L. et al. Quantifying the potential for reservoirs to secure future surface water yields in the world’s largest river basins. Environ. Res. Lett. 13 , 044026 (2018).
Aeschbach-Hertig, W. & Gleeson, T. Regional strategies for the accelerating global problem of groundwater depletion. Nat. Geosci. 5 , 853–861 (2012).
Gleeson, T., Cuthbert, M., Ferguson, G. & Perrone, D. Global groundwater sustainability, resources, and systems in the Anthropocene. Annu. Rev. Earth Planet. Sci. 48 , 431–463 (2020).
Wada, Y. & Bierkens, M. F. P. Sustainability of global water use: past reconstruction and future projections. Environ. Res. Lett. 9 , 104003 (2014).
Rodell, M., Velicogna, I. & Famiglietti, J. S. Satellite-based estimates of groundwater depletion in India. Nature 460 , 999–1002 (2009).
Wada, Y., van Beek, L. P. H. & Bierkens, M. F. P. Nonsustainable groundwater sustaining irrigation: a global assessment. Water Resour. Res. https://doi.org/10.1029/2011WR010562 (2012).
Famiglietti, J. S. The global groundwater crisis. Nat. Clim. Change 4 , 945–948 (2014).
Konikow, L. F. & Kendy, E. Groundwater depletion: a global problem. Hydrogeol. J. 13 , 317–320 (2005).
Siebert, S. et al. Groundwater use for irrigation—a global inventory. Hydrol. Earth Syst. Sci. 14 , 1863–1880 (2010).
Grogan, D. S., Wisser, D., Prusevich, A., Lammers, R. B. & Frolking, S. The use and re-use of unsustainable groundwater for irrigation: a global budget. Environ. Res. Lett. 12 , 034017 (2017).
Aquastat Database (FAO, accessed 5 June 2022); https://www.fao.org/nr/water/aquastat/data/query/index.html?lang=en
Dalin, C., Wada, Y., Kastner, T. & Puma, M. J. Groundwater depletion embedded in international food trade. Nature 543 , 700–704 (2017).
Gleeson, T., Wada, Y., Bierkens, M. F. P. & van Beek, L. P. H. Water balance of global aquifers revealed by groundwater footprint. Nature 488 , 197–200 (2012).
Dolan, F. et al. Evaluating the economic impact of water scarcity in a changing world. Nat. Commun. 12 , 1915 (2021).
Birnbaum, A., Lamontagne, J., Wild, T., Dolan, F. & Yarlagadda, B. Drivers of future physical water scarcity and its economic impacts in Latin America and the Caribbean. Earths Future 10 , e2022EF002764 (2022).
Seppelt, R., Manceur, A. M., Liu, J., Fenichel, E. P. & Klotz, S. Synchronized peak-rate years of global resources use. Ecol. Soc. 10.5751/ES-07039-190450 (2014).
Hubbert, M. K. Nuclear Energy and the Fossil Fuels Vol. 95 (Shell Development Company, Exploration and Production Research Division, 1956); http://www.energycrisis.com/Hubbert/1956/1956.pdf
Meinert, L. D., Robinson, G. R. & Nassar, N. T. Mineral resources: reserves, peak production and the future. Resources https://doi.org/10.3390/resources5010014 (2016).
Ericsson, M. & Söderholm, P. Mineral Depletion and Peak Production 222–231 (Palgrave Macmillan, 2013); https://doi.org/10.1057/9781137349149_12
Gleick, P. H. & Palaniappan, M. Peak water limits to freshwater withdrawal and use. Proc. Natl Acad. Sci. USA 107 , 11155–11162 (2010).
Turner, S. W. D., Hejazi, M., Yonkofski, C., Kim, S. H. & Kyle, P. Influence of groundwater extraction costs and resource depletion limits on simulated global nonrenewable water withdrawals over the twenty-first century. Earths Future 7 , 123–135 (2019).
Calvin, K. et al. Gcam v5.1: representing the linkages between energy, water, land, climate, and economic systems. Geosci. Model Dev. 12 , 677–698 (2019).
Gleeson, T. et al. The water planetary boundary: interrogation and revision. One Earth 2 , 223–234 (2020).
Graham, N. T. et al. Future changes in the trading of virtual water. Nat. Commun. 11 , 3632 (2020).
Riahi, K. et al. The shared socioeconomic pathways and their energy, land use, and greenhouse gas emissions implications: an overview. Glob. Environ. Change 42 , 153–168 (2017).
van Vuuren, D. P. et al. The representative concentration pathways: an overview. Clim. Change 109 , 5 (2011).
Scanlon, B. R. et al. Global models underestimate large decadal declining and rising water storage trends relative to GRACE satellite data. Proc. Natl Acad. Sci. USA 115 , E1080–E1089 (2018).
Perrone, D. & Jasechko, S. Deeper well drilling an unsustainable stopgap to groundwater depletion. Nat. Sustain. 2 , 773–782 (2019).
Gleeson, T., Befus, K. M., Jasechko, S., Luijendijk, E. & Cardenas, M. B. The global volume and distribution of modern groundwater. Nat. Geosci. 9 , 161–167 (2016).
de Graaf, I. E. M. et al. A global-scale two-layer transient groundwater model: development and application to groundwater depletion. Adv. Water Resour. 102 , 53–67 (2017).
Konikow, L. F. Contribution of global groundwater depletion since 1900 to sea-level rise. Geophys. Res. Lett. https://doi.org/10.1029/2011GL048604 (2011).
Wada, Y. et al. Global depletion of groundwater resources. Geophys. Res. Lett. https://doi.org/10.1029/2010GL044571 (2010).
Huang, Z. et al. Global agricultural green and blue water consumption under future climate and land use changes. J. Hydrol. 574 , 242–256 (2019).
Fitton, N. et al. The vulnerabilities of agricultural land and food production to future water scarcity. Glob. Environ. Change 58 , 101944 (2019).
Turner, S. W. D., Hejazi, M., Calvin, K., Kyle, P. & Kim, S. A pathway of global food supply adaptation in a world with increasingly constrained groundwater. Sci. Total Environ. 673 , 165–176 (2019).
van Vuuren, D. P. et al. The shared socio-economic pathways: trajectories for human development and global environmental change. Glob. Environ. Change 42 , 148–152 (2017).
O’Neill, B. C. et al. The roads ahead: narratives for shared socioeconomic pathways describing world futures in the 21st century. Glob. Environ. Change 42 , 169–180 (2017).
Graham, N. T. et al. Water sector assumptions for the shared socioeconomic pathways in an integrated modeling framework. Water Resour. Res. 54 , 6423–6440 (2018).
Hejazi, M. I. et al. Integrated assessment of global water scarcity over the 21st century under multiple climate change mitigation policies. Hydrol. Earth Syst. Sci. 18 , 2859–2883 (2014).
Hejazi, M. I. et al. 21st century United States emissions mitigation could increase water stress more than the climate change it is mitigating. Proc. Natl Acad. Sci. USA 112 , 10635–40 (2015).
Heistermann, M. Hess opinions: a planetary boundary on freshwater use is misleading. Hydrol. Earth Syst. Sci. 21 , 3455–3461 (2017).
Rockström, J. et al. Future water availability for global food production: the potential of green water for increasing resilience to global change. Water Resour. Res. https://doi.org/10.1029/2007WR006767 (2009).
Jägermeyr, J., Pastor, A., Biemans, H. & Gerten, D. Reconciling irrigated food production with environmental flows for sustainable development goals implementation. Nat. Commun. 8 , 15900 (2017).
D’Odorico, P. et al. The global food–energy–water nexus. Rev. Geophys. 56 , 456–531 (2018).
Wada, Y. et al. Modeling global water use for the 21st century: Water Futures and Solutions (WFAS) initiative and its approaches. Geosci. Model Dev. 9 , 175–222 (2016).
Byers, E. et al. Global exposure and vulnerability to multi-sector development and climate change hotspots. Environ. Res. Lett. 13 , 055012 (2018).
Miralles-Wilhelm, F. Water is the middle child in global climate policy. Nat. Clim. Change 12 , 110–112 (2022).
Gleick, P. H. Global freshwater resources: soft-path solutions for the 21st century. Science 302 , 1524–1528 (2003).
Zhao, X., Calvin, K. V., Wise, M. A. & Iyer, G. The role of global agricultural market integration in multiregional economic modeling: using hindcast experiments to validate an Armington model. Econ. Anal. Policy 72 , 1–17 (2021).
Bryant, B. P. & Lempert, R. J. Thinking inside the box: a participatory, computer-assisted approach to scenario discovery. Technol. Forecast. Soc. Change 77 , 34–49 (2010).
Gleeson, T. et al. GMD perspective: the quest to improve the evaluation of groundwater representation in continental- to global-scale models. Geosci. Model Dev. 14 , 7545–7571 (2021).
Wada, Y. et al. Human–water interface in hydrological modelling: current status and future directions. Hydrol. Earth Syst. Sci. 21 , 4169–4193 (2017).
Niazi, H. et al. Large ensemble dataset for discovering global peak water limit of future groundwater withdrawals using 900 GCAM runs. Zenodo https://doi.org/10.5281/zenodo.6480465 (2023).
Niazi, H. Meta-repository for groundwater peak and decline: JGCRI/niazi-etal_2024_nature-sustainability: v1-accepted. Zenodo https://doi.org/10.5281/zenodo.10524993 (2024).
Kim, S. H. et al. Balancing global water availability and use at basin scale in an integrated assessment model. Clim. Change 136 , 217–231 (2016).
Hejazi, M. et al. Long-term global water projections using six socioeconomic scenarios in an integrated assessment modeling framework. Technol. Forecast. Soc. Change 81 , 205–226 (2014).
Liu, Y., Hejazi, M., Li, H., Zhang, X. & Leng, G. A hydrological emulator for global applications—HE v1.0.0. Geosci. Model Dev. 11 , 1077–1092 (2018).
Vernon, C. R. et al. A global hydrologic framework to accelerate scientific discovery. J. Open Res. Softw. 10.5334/jors.245 (2019).
Richts, A., Struckmeier, W. F. & Zaepke, M. WHYMAP and the Groundwater Resources Map of the World 1:25,000,000 159–173 (Springer, 2011); https://doi.org/10.1007/978-90-481-3426-7_10
Gleeson, T., Moosdorf, N., Hartmann, J. & van Beek, L. P. H. A glimpse beneath earth’s surface: GLobal HYdrogeology MaPS (GLHYMPS) of permeability and porosity. Geophys. Res. Lett. 41 , 3891–3898 (2014).
de Graaf, I. E. M., Sutanudjaja, E. H., van Beek, L. P. H. & Bierkens, M. F. P. A high-resolution global-scale groundwater model. Hydrol. Earth Syst. Sci. 19 , 823–837 (2015).
Fan, Y., Li, H. & Miguez-Macho, G. Global patterns of groundwater table depth. Science 339 , 940–943 (2013).
Kyle, P. et al. Assessing the future of global energy-for-water. Environ. Res. Lett. 16 , 024031 (2021).
Cui, R. Y. et al. Regional responses to future, demand-driven water scarcity. Environ. Res. Lett. 13 , 094006 (2018).
Liu, L., Hejazi, M., Iyer, G. & Forman, B. A. Implications of water constraints on electricity capacity expansion in the United States. Nat. Sustain. 2 , 206–213 (2019).
Giuliani, M., Lamontagne, J. R., Hejazi, M. I., Reed, P. M. & Castelletti, A. Unintended consequences of climate change mitigation for African river basins. Nat. Clim. Change 12 , 187–192 (2022).
Lempert, R. J. A new decision sciences for complex systems. Proc. Natl Acad. Sci. USA 99 , 7309–7313 (2002).
Lamontagne, J. R. et al. Large ensemble analytic framework for consequence-driven discovery of climate change scenarios. Earths Future 6 , 488–504 (2018).
Warszawski, L. et al. The Inter-Sectoral Impact Model Intercomparison Project (ISI–MIP): project framework. Proc. Natl Acad. Sci. USA 111 , 3228–3232 (2014).
Turner, S. W., Ng, J. Y. & Galelli, S. Examining global electricity supply vulnerability to climate change using a high-fidelity hydropower dam model. Sci. Total Environ. 590 , 663–675 (2017).
Schewe, J. et al. Multimodel assessment of water scarcity under climate change. Proc. Natl Acad. Sci. USA 111 , 3245–3250 (2014).
Rosenzweig, C. et al. Assessing agricultural risks of climate change in the 21st century in a global gridded crop model intercomparison. Proc. Natl Acad. Sci. USA 111 , 3268–3273 (2014).
Clarke, L. et al. Effects of long-term climate change on global building energy expenditures. Energy Econ. 72 , 667–677 (2018).
Döll, P., Müller Schmied, H., Schuh, C., Portmann, F. T. & Eicker, A. Global‐scale assessment of groundwater depletion and related groundwater abstractions: combining hydrological modeling with information from well observations and GRACE satellites. Water Resour. Res. 50 , 5698–5720 (2014).
Hanasaki, N., Yoshikawa, S., Pokhrel, Y. & Kanae, S. A global hydrological simulation to specify the sources of water used by humans. Hydrol. Earth Syst. Sci. 22 , 789–817 (2018).
Pokhrel, Y. N. et al. Model estimates of sea-level change due to anthropogenic impacts on terrestrial water storage. Nat. Geosci. 5 , 389–392 (2012).
Pokhrel, Y. N. et al. Incorporation of groundwater pumping in a global Land Surface Model with the representation of human impacts. Water Resour. Res. 51 , 78–96 (2015).
van Dijk, A. I. J. M., Renzullo, L. J., Wada, Y. & Tregoning, P. A global water cycle reanalysis (2003–2012) merging satellite gravimetry and altimetry observations with a hydrological multi-model ensemble. Hydrol. Earth Syst. Sci. 18 , 2955–2973 (2014).
Wada, Y. Past and future contribution of global groundwater depletion to sea‐level rise. Geophys. Res. Lett. 39 , L09402 (2012).
Yoshikawa, S., Cho, J., Yamada, H. G., Hanasaki, N. & Kanae, S. An assessment of global net irrigation water requirements from various water supply sources to sustain irrigation: rivers and reservoirs (1960–2050). Hydrol. Earth Syst. Sci. 18 , 4289–4310 (2014).
Bierkens, M. F. P. & Wada, Y. Non-renewable groundwater use and groundwater depletion: a review. Environ. Res. Lett. 14 , 063002 (2019).
Download references
Acknowledgements
This research was supported by the U.S. Department of Energy, Office of Science, as part of research in MultiSector Dynamics of the Earth and Environmental System Modeling Program. The Pacific Northwest National Laboratory is operated for the DOE by Battelle Memorial Institute under contract DE-AC05-76RL01830. Authors M.H., S.T. and S.M. primarily contributed to this work while at the Pacific Northwest National Laboratory. The views and opinions expressed in this paper are those of the authors alone and should not be construed to represent any official USDA, USDOE or US Government determination or policy.
Author information
Authors and affiliations.
Joint Global Change Research Institute, Pacific Northwest National Laboratory, College Park, MD, USA
Hassan Niazi, Thomas B. Wild, Neal T. Graham & Son Kim
Oak Ridge National Laboratory, Oak Ridge, TN, USA
Sean W. D. Turner
King Abdullah Petroleum Studies and Research Center, Riyadh, Saudi Arabia
Mohamad Hejazi
Economic Research Service, United States Department of Agriculture, Washington DC, USA
Siwa Msangi
Tufts University, Medford, MA, USA
Jonathan R. Lamontagne
Pacific Northwest National Laboratory, Richland, WA, USA
Mengqi Zhao
You can also search for this author in PubMed Google Scholar
Contributions
All authors contributed to this study. M.H. and S.T. conceptualized the study. H.N., S.T., T.W. and N.G. conducted modelling and analysis, including designing scenario experiments, analysing GCAM modelling outputs and visualizing results, and all authors contributed towards the writing of the paper.
Corresponding author
Correspondence to Hassan Niazi .
Ethics declarations
Competing interests.
The authors declare no competing interests.
Peer review
Peer review information.
Nature Sustainability thanks Marc Bierkens, Inge de Graaf and the other, anonymous, reviewer(s) for their contribution to the peer review of this work.
Additional information
Publisher’s note Springer Nature remains neutral with regard to jurisdictional claims in published maps and institutional affiliations.
Supplementary information
Supplementary information.
Supplementary Figs. 1–24, Supplementary Tables 1–8, equations 1–4, 44 additional literature references and links to a publicly accessible meta-repository of data and code: https://github.com/JGCRI/niazi-etal_2024_nature-sustainability .
Reporting Summary
Rights and permissions.
Springer Nature or its licensor (e.g. a society or other partner) holds exclusive rights to this article under a publishing agreement with the author(s) or other rightsholder(s); author self-archiving of the accepted manuscript version of this article is solely governed by the terms of such publishing agreement and applicable law.
Reprints and permissions
About this article
Cite this article.
Niazi, H., Wild, T.B., Turner, S.W.D. et al. Global peak water limit of future groundwater withdrawals. Nat Sustain 7 , 413–422 (2024). https://doi.org/10.1038/s41893-024-01306-w
Download citation
Received : 24 May 2023
Accepted : 06 February 2024
Published : 22 April 2024
Issue Date : April 2024
DOI : https://doi.org/10.1038/s41893-024-01306-w
Share this article
Anyone you share the following link with will be able to read this content:
Sorry, a shareable link is not currently available for this article.
Provided by the Springer Nature SharedIt content-sharing initiative
Quick links
- Explore articles by subject
- Guide to authors
- Editorial policies
Sign up for the Nature Briefing: Anthropocene newsletter — what matters in anthropocene research, free to your inbox weekly.

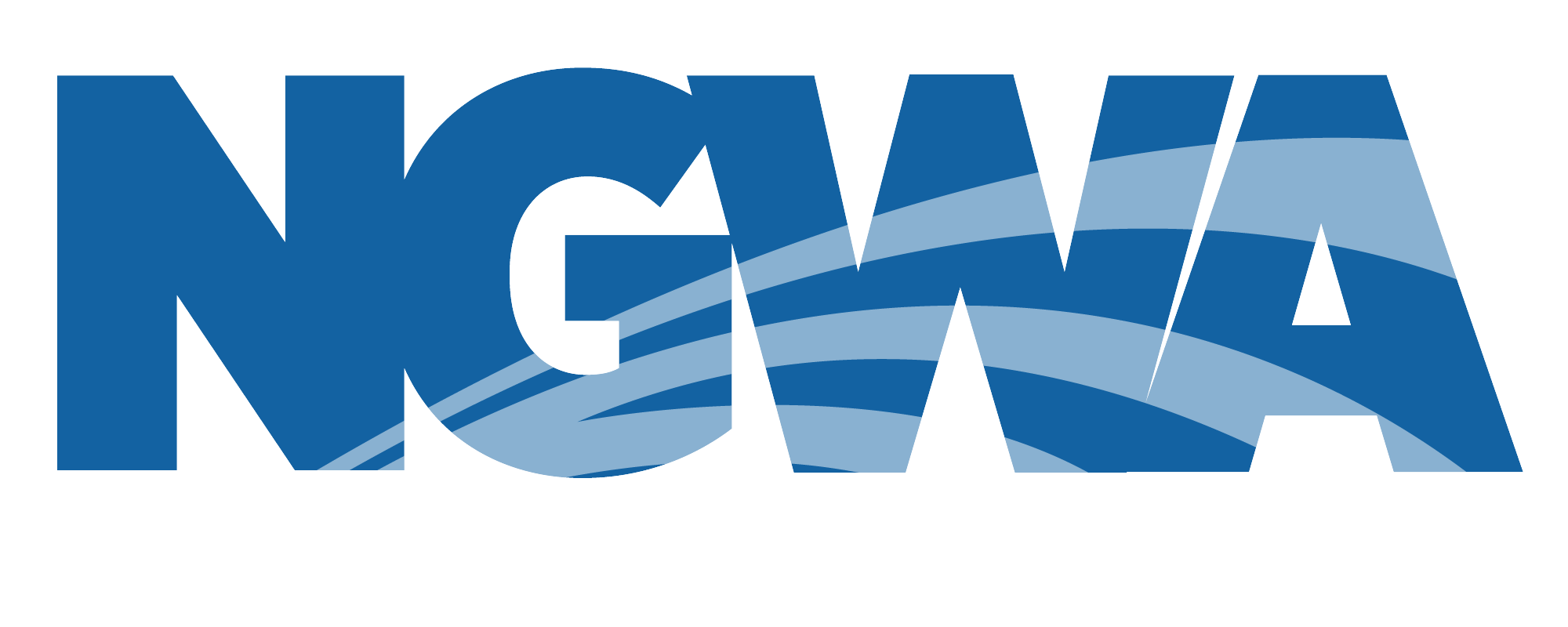
- Prepare a Research Paper for Groundwater
- Submit a Paper for Publication
- Peer Review Process
- Types of Articles Published in Groundwater
- Submission Policies
- Open Access
- Volunteer to be a Reviewer
Topics on any subject pertaining to groundwater will be considered for publication.
Manuscripts must not have been previously published or currently submitted elsewhere for publication while in review for Groundwater ® .
See an example Groundwater manuscript.
Articles “prepublished” as preprints or in similar “early-submission repositories” can be submitted to Groundwater . If the article has been rejected for formal publication elsewhere, Groundwater will only accept a substantially revised version of the prepublished manuscript. Mention of prepublication and manuscript status must be made at time of submission. If accepted and published in Groundwater , a special footnote will be added which mentions the repository the preprint is housed in as well as the preprint’s DOI number.
Papers of several different types are published in Groundwater . Please consult the definitions of paper types. Also please note that various paper types have different limits on paper length and other specific requirements.
Manuscripts and all figures must be in an electronic format in order to utilize our online review system. Manuscripts should be prepared in a word processing program, such as Microsoft Word, double spaced in size 12-point font, with line numbering.
- Manuscripts must be in English.
- Title should be fewer than 100 characters (including punctuation and spaces).
- Abstract should contain fewer than 250 words.
- The article impact statement should be no more than 140 characters (including punctuation and spaces).
- Number pages consecutively starting with the title page.
- Use the “line numbering” feature in your word processing program.
Units of measurements
- Abbreviations, symbols, units, and Greek letters should be identified the first time they are used.
- Isotopic data should be expressed following the guidelines set forth in Ground Water 34, no. 3, page 388 (1996). See the guidelines (PDF).
- Either metric, SI, or U.S. customary (English) units may be used. If U.S. customary units are used, the author is asked to provide the conversion to metric/SI units in parenthesis when units are given in the text of the paper. It is not necessary to give the conversions in tables and figures.
Acknowledgments
Acknowledgments should be made only for significant contributions by professional associates, permission to publish by employer, access to land or equipment, financial support, and reviews. A brief closing statement will usually suffice.
- Indicate in text by author(s) name and year (e.g., Jones 1999). For multiple references by the same author in the same year, the letters a, b, and c after the year can be used (e.g., Jones 1999a and 1999b). Papers with multiple authors should be cited in the text using “et al.” (e.g., Jones et al. 1999).
- At the end of the paper, list alphabetically by author(s) name in chronological order.
- Write in the style of the Harvard referencing style. View reference samples.
- Do not reference limited access, administrative, or confidential reports or commercial products for which a charge will be made for access.
- Include personal communication references (written or oral) in the reference list.
- Do not abbreviate publication titles.
- We require that journal papers be cited using both volume and issue number (e.g., Groundwater 32, no. 4: 257-269).
- Do not use footnotes. (Footnotes are acceptable in tables only.)
It is highly recommended that authors utilize some of the many online search engines to ensure that all publications relevant to their research are identified.
Supporting information
Supporting information is important, ancillary information relevant to the article that does not appear in the print or online version of the journal. It can comprise additional tables, data sets, figures, movie files, audio clips, 3-D structures, and other related nonessential multimedia files. Read more detail from Wiley on supporting information.
Should be numbered and titled. Tables should be embedded “inline” in the manuscript.
- Figures should be prepared at the final publication size (8.25 cm wide for one column and 17.15 cm wide for two columns). It is a good idea to prepare your figures with final publication requirements in mind, and then convert them into formats appropriate for online review.
- Color figures are published free of charge to the author. However, as there are additional costs to print color, it is asked that the author use color figures only when necessary to improve the clarity of the figure.
- Digital figures are required. Digital figures must have a resolution of at least 300 dpi (dots per inch) and be submitted in a TIF or EPS format with embedded fonts.
- Always submit the image at its final size. For Groundwater , that is 8.25 cm wide for one-column art and 17.15 cm wide for two-column art.
- Generate the image at line screens of 85 lines per inch (lpi) or lower.
- When applying multiple shades of gray, differentiate the gray levels by at least 20 percent. In other words, apply gray in increments or steps no closer than 20 percent. Use 20 percent black, 40 percent black, and 60 percent black. Alternatively, use various pattern backgrounds (solid dots, lines, bricks, diamonds, squares, etc.).
- Never use levels of gray below 20 percent or above 70 percent black. Levels of gray outside these guidelines will either fade out or become totally black upon printing.
- Use thick, solid lines that are no finer than 1 point in thickness.
- Use bold, solid type. Avoid using type with serifs (e.g., Times); sans serif type (e.g., Helvetica) reproduces best.
- At 100 percent size, no type should be smaller than 5 point.
- Avoid layering type directly over shaded or textured areas. Create a white box and place the lettering within the boundaries of the white box.
- Avoid the use of reversed type (white lettering on a black background).
- When scanning images, be sure that the originals are perfectly clean and neat. Scanners faithfully reproduce all smudges, crooked lines, and stray marks.
- When printing images that will be scanned, use high-quality laser printer paper or bond paper. Do not use photocopies. Opacity and smoothness are important quality factors in the scanning process. Use the highest resolution possible on your printer or graphics plotting device.
- When preparing figures that will be scanned, remember that surface smoothness is critical. Tape, creases, and other minor surface irregularities are unacceptable since they will produce shadows that the scanner sees as black. Maximize the black and white contrast (the toner level in the printing device must be high). The scanner does not improve contrast; it reproduces contrast as is.
- Color publication is available. Color figures are published free of charge to the author. However, as there are additional costs to print color, it is asked that the author use color figures only when necessary to improve the clarity of the figure.
Embedded Rich Media
The journal facilitates and encourages embedded rich media as part of a submitted paper. For specifications as to accepted file types and file size see: Embedded Rich Media .
Embedded rich media that includes speech must be accompanied by a transcript. This must be provided under the file designation of "Transcription". If the speech is not English, an English translation of the transcript must also be provided in the same file. Each embedded media file must be numbered (e.g. Audio 1, Audio 2, etc) as figures or tables would be. This numbering must be reflected in the manuscript (i.e. where the rich media is cited in the body text of the manuscript) and in the legend (e.g. "Video 1: Demonstration of Technique"). For every video file there should also be a placeholder image, which would usually be a still image taken from the video. This should be uploaded with the file designation "Placeholder Image (Video Only)", as well as the timestamp in seconds.
Graphical Abstract
A Graphical Abstract is a single, concise visual summary of the main topics or findings of the article. The journal allows authors the option of submitting a Graphical Abstract with all article types that have a written abstract. Upon publication, the Graphical Abstract will be displayed next to the published article within the Table of Contents on Wiley Online Library.
The Graphical Abstract should be a single-page illustration or graphic image that gives readers a visual depiction of the article’s “take-away” message. The image may be a figure or image included in the text itself, or a new figure generated specifically for this purpose.
Requirements include the following:
- The submitted image should be 5.5 inches square at 300 dpi
- Preferred file types — TIFF, PDF, JPG
- A brief one- to two-sentence summary of the key findings presented in the paper.
Before you submit your paper, please gather the following information
- Author information. The first and last name and affiliation of all authors, and the postal address, telephone and fax numbers, and email address of the corresponding author. The person who uploads the manuscript must be the corresponding author.
- Title and abstract of the paper. You can copy and paste this from your manuscript.
- Paper type (e.g., research paper, issue paper) . See the definitions of the different paper types.
- Manuscript files in Microsoft Word or PDF.
- It is recommended that the manuscript contains the tables and figures embedded “inline.” This way, the author has to upload only one document. Figures can be uploaded separately, if desired.
- Figures at this stage do not have to be publication quality, but it is a good idea to start with publication-quality figures and then save them in the formats allowed by the system.
Development of anthropogenic water regulation for Community integrated Earth System model (CIESM)
- Zhan, Chesheng
- Zhang, Haoyue
- Li, Zhonghe
This study examines the impact of anthropogenic water regulation (AWR) on hydroclimatic systems by incorporating an AWR module into the Community Integrated Earth System Model (CIESM), validated against GRACE satellite data. This approach assesses the influence of human activities, including irrigation and groundwater extraction, on global and regional hydrology and climate. Key findings include: Implementation of the AWR module significantly improves terrestrial water storage (TWS) simulation accuracy in CIESM. The correlation coefficient for global-scale TWS improved from 0.33 in the control (CTRL) to 0.89 in the experiment (EXP). Notably, the model's performance enhanced in Northern India and the North China Plain, while the Central United States showed limited improvement. AWR markedly alters the global water cycle, evidenced by substantial increases in soil moisture (about 0.04 to 0.02 m3/m3) and evapotranspiration. These changes have led to increased latent heat flux (around 5 W/m2) and a decrease in temperature by 0.1 °C to 1 °C in heavily irrigated regions. The study highlights the role of AWR in modifying the energy balance, particularly in agricultural areas where irrigation exerts a significant cooling effect. Despite its potential, the study identifies considerable uncertainties in coupling AWR within the CIESM model, related to inherent model limitations, incomplete water intake data, variable groundwater levels, and simplifications in water transfer parameters. Future research should aim to refine these aspects, focusing on enhancing the physical mechanisms and performance of AWR modules in hydroclimatic simulations. In conclusion, this research underscores the substantial modifications in hydrological and climatic conditions due to human activities. The improved AWR scheme within CIESM provides valuable insights for understanding and predicting climate change impacts on water resources, demonstrating its utility in simulating complex hydroclimatic changes at both global and regional scales.
- Anthropogenic water regulation;
- Water cycle;
- Model improvement
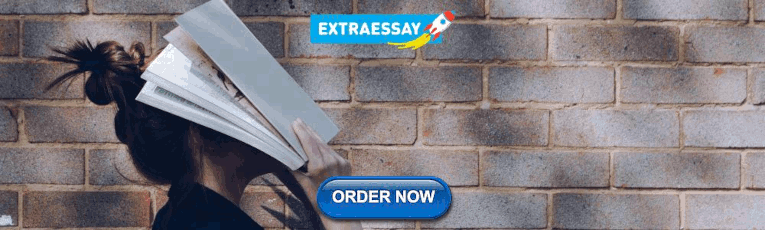
IMAGES
VIDEO
COMMENTS
There is much ongoing research on groundwater in LKHRs, and it needs to further expand and accelerate in support of global groundwater modeling needs. Of particular importance is the nature of the hydrogeologic transition from the uplands to the lowlands which is commonly referred to as the "mountain front" (Wilson & Guan, 2004).
1. Introduction. Groundwater is the largest freshwater store on earth, its use underpins a huge range of human activities as well as important ecosystems (Margat and Van der Gun, 2013; Rohde et al., 2017).Historically, groundwater quantity has often been the focus of groundwater resource assessments, and there is a real need to now focus more attention towards groundwater quality.
Groundwater consists. both of water that remains in the unsaturated or. vadose zone (also often termed "soil water") and. of water that reaches the saturated zone (aquifer) where pore spaces ...
This review paper is organized into five sections: (1) a review of the global climate change; (2) an assessment of the present state of climate change impact on groundwater components; (3) a review of groundwater models and climate change induced future groundwater changes; (4) groundwater feedbacks to the climate system; and (5) key ...
National Centre for Groundwater Research and Training (NCGRT), College of Science and Engineering, Flinders University, Adelaide, South Australia, Australia ... All authors reviewed the paper and ...
Global groundwater withdrawals were estimated to have exceeded 900 km 3 /year by 2010, with water wells and springheads providing some 36% of potable water supply (60). Groundwater, the world's largest distributed store of fresh water, is naturally well placed to play a vital role in enabling societies to adapt to intermittent and sustained water shortages caused by climate change.
Groundwater, the vast water reserve beneath Earth's surface 1, is an essential resource for humans and ecosystems.Globally, more than one-third of the water used originates from underground 2.In ...
Here we analyse in situ groundwater-level trends for 170,000 monitoring wells and 1,693 aquifer systems in countries that encompass approximately 75% of global groundwater withdrawals 18. We show ...
Groundwater® is the leading international journal focused exclusively on groundwater.Since 1963, Groundwater has published a dynamic mix of papers on topics related to groundwater including groundwater flow and well hydraulics, hydrogeochemistry and contaminant hydrogeology, application of geophysics, groundwater management and policy, and history of groundwater hydrology.
• Interdisciplinary information exchange and to stimulate scientific research in the fields of groundwater related sciences and social and health sciences required to achieve the United Nations Millennium Development Goals for sustainable development. ... A bibliometric analysis of scopus-based papers from 1972 to 2023, emphasizing the ...
Groundwater contamination is a global problem that has a significant impact on human health and ecological services. Studies reported in this special issue focus on contaminants in groundwater of geogenic and anthropogenic origin distributed over a wide geographic range, with contributions from researchers studying groundwater contamination in India, China, Pakistan, Turkey, Ethiopia, and ...
Groundwater comprises 95% of the liquid fresh water on Earth and contains a diverse mix of dissolved organic matter (DOM) molecules which play a significant role in the global carbon cycle.
Groundwater is a vital and purest form of natural resource. In the recent years, various anthropogenic causes threat its natural quality. Therefore, its suitability for drinking, irrigation and other purposes make doubtful conditions of human well-being, especially in developing countries. In this present study, groundwater quality was evaluated for drinking, irrigation and human health hazard ...
1. Introduction. Earth is known as the blue planet or the water planet because of the reality that most of its surface is covered by water, and it is the only planet in the solar system that has this huge quantity of water [1,2].For various authorities and agencies dealing with water problems, the conservation of surface and groundwater purity without pollution is indeed an aim.
Groundwater is an essential resource for man's survival and is imperative for public health [1].Statistically, groundwater constitutes 97% of the global freshwater and is a major drinking water source and a critical resort for water resources for domestic and public use [[2], [5]].Besides, it is a precious resource in arid areas due to erratic rainfall and limited surface water resources [3].
The paper concluded by recommending research into quantifying groundwater, its quality and treatment based on the above overview. Drinking water quality. Taste threshold for major cations.
Chapter - 10. Geochemistry of Groundwater. Vinuta M. Betageri and Rahul Patil. Abstract. There is scope f or groundwater assessment, encompassing a range of. quality issues, purposes, types ...
Groundwater is an important source for drinking water supply in hard rock terrain of Bundelkhand massif particularly in District Mahoba, Uttar Pradesh, India. An attempt has been made in this work to understand the suitability of groundwater for human consumption. The parameters like pH, electrical conductivity, total dissolved solids, alkalinity, total hardness, calcium, magnesium, sodium ...
Over the past 50 years, humans have extracted the Earth's groundwater stocks at a steep rate, largely to fuel global agro-economic development. Given society's growing reliance on groundwater ...
Groundwater related research papers from 1978 to 2017 were collected. • The number of published studies increased by >10% per year on average. • Institutions based in developed countries have led groundwater research output. • China and India have experienced strong growth in output in recent years. •
Always submit the image at its final size. For Groundwater, that is 8.25 cm wide for one-column art and 17.15 cm wide for two-column art. Generate the image at line screens of 85 lines per inch (lpi) or lower. When applying multiple shades of gray, differentiate the gray levels by at least 20 percent.
1- Groundwater Nitrate Contamination: Inorganic contamination of the greatest concern in groundwater. is nitrate ions, which usually occur in aquifers near rural and suburban populations. Although ...
1.Introduction. Groundwater in upland landscapes regulates baseflow, providing a vital water resource for irrigation, potable water, and ecological functions (Dralle et al., 2023).In developing countries, dug wells and springs fed by hillslope aquifers are often the sole water source (Awulachew and Ayana, 2011, Yimam et al., 2021) and are essential in poverty alleviation, food security, and ...
This study examines the impact of anthropogenic water regulation (AWR) on hydroclimatic systems by incorporating an AWR module into the Community Integrated Earth System Model (CIESM), validated against GRACE satellite data. This approach assesses the influence of human activities, including irrigation and groundwater extraction, on global and regional hydrology and climate.
1. Introduction. Freshwater is a limited natural resource that sustains both ecosystems and human societies. Rivers, lakes, groundwater, etc. are the major sources of freshwater, which covers only 2.5% of water on the earth's surface (Akter et al., 2018, Ghosh et al., 2022, Sajib et al., 2023).Among them, groundwater is considered a significant source of potable water for human consumption ...