Metal-organic frameworks: Synthetic methods for industrial production
- Review Article
- Published: 10 March 2023
- Volume 16 , pages 7906–7925, ( 2023 )
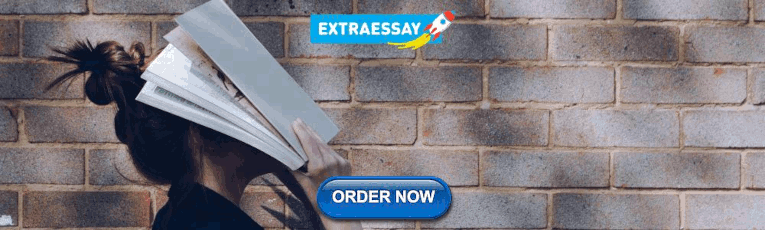
Cite this article
- Dou Ma 1 , 2 ,
- Xin Huang 1 ,
- Yu Zhang 3 ,
- Lu Wang 1 &
- Bo Wang 1 , 2
1157 Accesses
14 Citations
1 Altmetric
Explore all metrics
Metal-organic frameworks (MOFs), which are constructed by metal ions or clusters with organic ligands, have shown great potential in gas storage and separation, luminescence, catalysis, drug delivery, sensing, and so on. More than 20,000 MOFs have been reported by adjusting the composition and reaction conditions, and most of them were synthesized by hydrothermal or solvothermal methods. The conventional solvothermal methods are favorable for the slow crystallization of MOFs to obtain single crystals or highly crystalline powders, which are suitable for the structure analysis. However, their harsh synthesis conditions, long reaction time, and difficulty in continuous synthesis limit their scale-up in industrial production and application. Meanwhile, shaping or processing is also required to bring MOF crystals and powders into the market. Therefore, this review demonstrates the crystallization mechanisms of MOFs to understand how the synthetic parameters affect the final products. Additionally, a variety of promising synthetic routes which can be used for large scale synthesis were reviewed in details. Lastly, the prospects of MOF shaping and processing are provided to promote their industrial application.
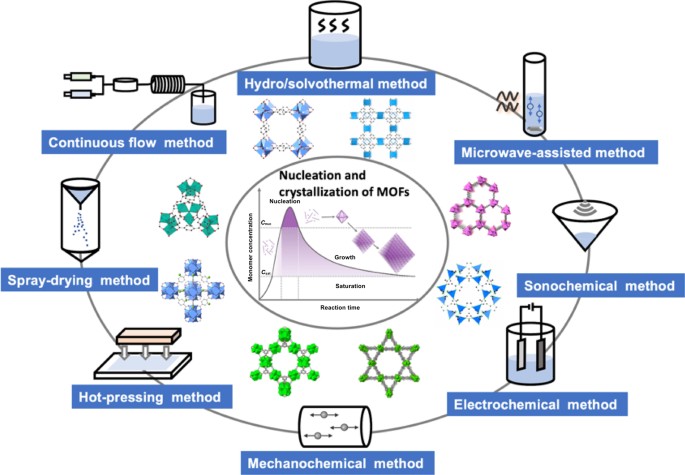
This is a preview of subscription content, log in via an institution to check access.
Access this article
Price includes VAT (Russian Federation)
Instant access to the full article PDF.
Rent this article via DeepDyve
Institutional subscriptions
Similar content being viewed by others
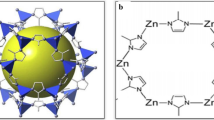
A review on zeolite imidazole frameworks: synthesis, properties, and applications
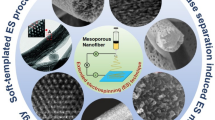
Mesoporous Nanofibers from Extended Electrospinning Technique
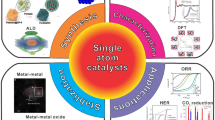
Single-Atom Catalysts: From Design to Application
Getman, R. B.; Bae, Y. S.; Wilmer, C. E.; Snurr, R. Q. Review and analysis of molecular simulations of methane, hydrogen, and acetylene storage in metal-organic frameworks. Chem. Rev. 2012 , 112 , 703–723.
CAS Google Scholar
Murray, L. J.; Dincă, M.; Long, J. R. Hydrogen storage in metal-organic frameworks. Chem. Soc. Rev. 2009 , 38 , 1294–1314.
Adil, K.; Belmabkhout, Y.; Pillai, R. S.; Cadiau, A.; Bhatt, P. M.; Assen, A. H.; Maurin, G.; Eddaoudi, M. Gas/vapour separation using ultra-microporous metal-organic frameworks: Insights into the structure/separation relationship. Chem. Soc. Rev. 2017 , 46 , 3402–3430.
Lin, R. B.; Xiang, S. C.; Xing, H. B.; Zhou, W.; Chen, B. L. Exploration of porous metal-organic frameworks for gas separation and purification. Coord. Chem. Rev. 2019 , 378 , 87–103.
Cadiau, A.; Adil, K.; Bhatt, P. M.; Belmabkhout, Y.; Eddaoudi, M. A metal-organic framework-based splitter for separating propylene from propane. Science 2016 , 353 , 137–140.
Allendorf, M. D.; Bauer, C. A.; Bhakta, R. K.; Houk, R. J. T. Luminescent metal-organic frameworks. Chem. Soc. Rev. 2009 , 38 , 1330–1352.
Yang, Q. H.; Xu, Q.; Jiang, H. L. Metal-organic frameworks meet metal nanoparticles: Synergistic effect for enhanced catalysis. Chem. Soc. Rev. 2017 , 46 , 4774–4808.
Xiao, J. D.; Jiang, H. L. Metal-organic frameworks for photocatalysis and photothermal catalysis. Acc. Chem. Res. 2019 , 52 , 356–366.
Sui, J.; Liu, H.; Hu, S. J.; Sun, K.; Wan, G.; Zhou, H.; Zheng, X.; Jiang, H. L. A general strategy to immobilize single-atom catalysts in metal-organic frameworks for enhanced photocatalysis. Adv. Mater. 2022 , 34 , 2109203.
Velásquez-Hernández, M. D. J.; Linares-Moreau, M.; Astria, E.; Carraro, F.; Alyami, M. Z.; Khashab, N. M.; Sumby, C. J.; Doonan, C. J.; Falcaro, P. Towards applications of bioentities@MOFs in biomedicine. Coord. Chem. Rev. 2021 , 429 , 213651.
Google Scholar
Liang, W. B.; Wied, P.; Carraro, F.; Sumby, C. J.; Nidetzky, B.; Tsung, C. K.; Falcaro, P.; Doonan, C. J. Metal-organic framework-based enzyme biocomposites. Chem. Rev. 2021 , 121 , 1077–1129.
Peralta, R. A.; Huxley, M. T.; Evans, J. D.; Fallon, T.; Cao, H. J.; He, M. X.; Zhao, X. S.; Agnoli, S.; Sumby, C. J.; Doonan, C. J. Highly active gas phase organometallic catalysis supported within metal-organic framework pores. J. Am. Chem. Soc. 2020 , 142 , 13533–13543.
Lustig, W. P.; Mukherjee, S.; Rudd, N. D.; Desai, A. V.; Li, J.; Ghosh, S. K. Metal-organic frameworks: Functional luminescent and photonic materials for sensing applications. Chem. Soc. Rev. 2017 , 46 , 3242–3285.
Lamer, V. K.; Dinegar, R. H. Theory, production and mechanism of formation of monodispersed hydrosols. J. Am. Chem. Soc. 1950 , 72 , 4847–4854.
Feng, L.; Wang, K. Y.; Powell, J.; Zhou, H. C. Controllable synthesis of metal-organic frameworks and their hierarchical assemblies. Matter 2019 , 1 , 801–824.
Sosso, G. C.; Chen, J.; Cox, S. J.; Fitzner, M.; Pedevilla, P.; Zen, A.; Michaelides, A. Crystal nucleation in liquids: Open questions and future challenges in molecular dynamics simulations. Chem. Rev. 2016 , 116 , 7078–7116.
Mullin, J. W. Crystallization , 4th ed.; Butterworth-Heinemann: Oxford, 2001.
Van Vleet, M. J.; Weng, T. T.; Li, X. Y.; Schmidt, J. R. In situ , time-resolved, and mechanistic studies of metal-organic framework nucleation and growth. Chem. Rev. 2018 , 118 , 3681–3721.
Karthika, S.; Radhakrishnan, T. K.; Kalaichelvi, P. A review of classical and nonclassical nucleation theories. Cryst. Growth Des. 2016 , 16 , 6663–6681.
Xu, H. Q.; Wang, K. C.; Ding, M. L.; Feng, D. W.; Jiang, H. L.; Zhou, H. C. Seed-mediated synthesis of metal-organic frameworks. J. Am. Chem. Soc. 2016 , 138 , 5316–5320.
Liu, S. Y.; Zhang, Y.; Meng, Y.; Gao, F.; Jiao, S. J.; Ke, Y. C. Fast syntheses of MOFs using nanosized zeolite crystal seeds in situ generated from microsized zeolites. Cryst. Growth Des. 2013 , 13 , 2697–2702.
Mitsuka, Y.; Nagashima, K.; Kobayashi, H.; Kitagawa, H. A seed-mediated spray-drying method for fcile syntheses of Zr-MOF and a pillared-layer-type MOF. Chem. Lett. 2016 , 45 , 1313–1315.
Lim, I. H.; Schrader, W.; Schüth, F. Insights into the molecularassembly of zeolitic imidazolate frameworks by ESI-MS. Chem. Mater. 2015 , 27 , 3088–3095.
Patterson, J. P.; Abellan, P.; Denny, M. S. Jr.; Park, C.; Browning, N. D.; Cohen, S. M.; Evans, J. E.; Gianneschi, N. C. Observing the growth of metal-organic frameworks by in situ liquid cell transmission electron microscopy. J. Am. Chem. Soc. 2015 , 137 , 7322–7328.
Liu, X. W.; Chee, S. W.; Raj, S.; Sawczyk, M.; Král, P.; Mirsaidov, U. Three-step nucleation of metal-organic framework nanocrystals. Proc. Natl. Acad. Sci. USA 2021 , 118 , e2008880118.
Xing, J. F.; Schweighauser, L.; Okada, S.; Harano, K.; Nakamura, E. Atomistic structures and dynamics of prenucleation clusters in MOF-2 and MOF-5 syntheses. Nat. Commun. 2019 , 10 , 3608.
Burton, W. K.; Cabrera, N.; Frank, F. C. The growth of crystals and the equilibrium structure of their surfaces. Philos. Trans. Roy. Soc. Ser. A Mathemat. Phys. Sci. 1951 , 243 , 299–358.
Cubillas, P.; Anderson, M. W. Zeolites and Catalysis ; Wiley-VCH Verlag GmbH & Co. KGaA, 2010; pp 1–55.
Lovette, M. A.; Browning, A. R.; Griffin, D. W.; Sizemore, J. P.; Snyder, R. C.; Doherty, M. F. Crystal shape engineering. Ind. Eng. Chem. Res. 2008 , 47 , 9812–9833.
Van Santen, R. A. The ostwald step rule. J. Phys. Chem. 1984 , 88 , 5768–5769.
Voorhees, P. W. The theory of ostwald ripening. J. Stat. Phys. 1985 , 38 , 231–252.
Venna, S. R.; Jasnski, J. B.; Carreon M. A. Structural evolution of zeolitic imidazolate framework-8. J. Am. Chem. Soc. 2010 , 132 , 18030–18033.
De Yoreo, J. J.; Gilbert, P. U. P. A.; Sommerdijk, N. A. J. M.; Penn, R. L.; Whitelam, S.; Joester, D.; Zhang, H. Z.; Rimer, J. D.; Navrotsky, A.; Banfield, J. F. et al. Crystallization by particle attachment in synthetic, biogenic, and geologic environments. Science 2015 , 349 , aaa6760.
Han, J. L.; He, X. D.; Liu, J.; Ming, R. J.; Lin, M. H.; Li, H.; Zhou, X. C.; Deng, H. X. Determining factors in the growth of MOF single crystals unveiled by in situ interface imaging. Chem 2022 , 8 , 1637–1657.
Seoane, B.; Castellanos, S.; Dikhtiarenko, A.; Kapteijn, F.; Gascon, J. Multi-scale crystal engineering of metal organic frameworks. Coord. Chem. Rev. 2016 , 307 , 147–187.
Chin, J. M.; Chen, E. Y.; Menon, A. G.; Tan, H. Y.; Hor, A. T. S.; Schreyer, M. K.; Xu, J. W. Tuning the aspect ratio of NH 2 -MIL-53(Al) microneedles and nanorodsvia coordination modulation. CrystEngComm 2013 , 15 , 654–657.
Cravillon, J.; Nayuk, R.; Springer, S.; Feldhoff, A.; Huber, K.; Wiebcke, M. Controlling zeolitic imidazolate framework nano- and microcrystal formation: Insight into crystal growth by time-resolved in situ static light scattering. Chem. Mater. 2011 , 23 , 2130–2141.
Schaate, A.; Roy, P.; Godt, A.; Lippke, J.; Waltz, F.; Wiebcke, M.; Behrens, P. Modulated synthesis of Zr-based metal-organic frameworks: From nano to single crystals. Chem. —Eur. J. 2011 , 17 , 6643–6651.
Bentz, K. C.; Ayala, S.; Kalaj, M.; Cohen, S. M.; Bentz, K. C.; Ayala, S.; Kalaj, M.; Cohen, S. M. Polyacids as modulators for the synthesis of UiO-66. Aust. J. Chem. 2019 , 72 , 848–851.
Gong, X.; Shu, Y. F.; Jiang, Z.; Lu, L. X.; Xu, X. H.; Wang, C.; Deng, H. X. Metal-organic frameworks for the exploitation of distance between active sites in efficient photocatalysis. Angew. Chem., Int. Ed. 2020 , 59 , 5326–5331.
Liu, X. Y.; Liu, B.; Li, G. H.; Liu, Y. L. Two anthracene-based metal-organic frameworks for highly effective photodegradation and luminescent detection in water. J. Mater. Chem. A 2018 , 6 , 17177–17185.
Henkelis, S. E.; Vornholt, S. M.; Cordes, D. B.; Slawin, A. M. Z.; Wheatley, P. S.; Morris, R. E. A single crystal study of CPO-27 and UTSA-74 for nitric oxide storage and release. CrystEngComm 2019 , 21 , 1857–1861.
Umemura, A.; Diring, S.; Furukawa, S.; Uehara, H.; Tsuruoka, T.; Kitagawa, S. Morphology design of porous coordination polymer crystals by coordination modulation. J. Am. Chem. Soc. 2011 , 133 , 15506–15513.
Chen, Y. F.; Zhang, S. H.; Chen, F.; Cao, S. J.; Cai, Y.; Li, S. Q.; Ma, H. W.; Ma, X. J.; Li, P. F.; Huang, X. Q. et al. Defect engineering of highly stable lanthanide metal-organic frameworks by particle modulation for coating catalysis. J. Mater. Chem. A 2018 , 6 , 342–348.
Wu, H.; Chua, Y. S.; Krungleviciute, V.; Tyagi, M.; Chen, P.; Yildirim, T.; Zhou, W. Unusual and highly tunable missing-linker defects in zirconium metal-organic framework UiO-66 and their important effects on gas adsorption. J. Am. Chem. Soc. 2013 , 135 , 10525–10532.
Cai, G. R.; Jiang, H. L. A modulator-induced defect-formation strategy to hierarchically porous metal-organic frameworks with high stability. Angew. Chem., Int. Ed. 2017 , 56 , 563–567.
Ma, X.; Wang, L.; Zhang, Q.; Jiang, H. L. Switching on the photocatalysis of metal-organic frameworks by engineering structural defects. Angew. Chem., Int. Ed. 2019 , 58 , 12175–12179.
Feng, X.; Hajek, J.; Jena, H. S.; Wang, G. B.; Veerapandian, S. K. P.; Morent, R.; De Geyter, N.; Leyssens, K.; Hoffman, A. E. J.; Meynen, V. et al. Engineering a highly defective stable UiO-66 with tunable Lewis-Bronsted acidity: The role of the hemilabile linker. J. Am. Chem. Soc. 2020 , 142 , 3174–3183.
Stock, N.; Biswas, S. Synthesis of metal-organic frameworks (MOFs): Routes to various MOF topologies, morphologies, and composites. Chem. Rev. 2012 , 112 , 933–969.
Howarth, A. J.; Peters, A. W.; Vermeulen, N. A.; Wang, T. C.; Hupp, J. T.; Farha, O. K. Best practices for the synthesis, activation, and characterization of metal-organic frameworks. Chem. Mater. 2017 , 29 , 26–39.
Cohen, S. M. Postsynthetic methods for the functionalization of metal-organic frameworks. Chem. Rev. 2012 , 112, 970–1000.
Rabenau, A. The role of hydrothermal synthesis in preparative chemistry. Angew. Chem., Int. Ed. 1985 , 24 , 1026–1040.
Burrows, A. D.; Cassar, K.; Düren, T.; Friend, R. M.; Mahon, M. F.; Rigby, S. P.; Savarese, T. L. Syntheses, structures and properties of cadmium benzenedicarboxylate metal-organic frameworks. Dalton Trans. 2008 , 2465–2474.
Rowsell, J. L. C.; Yaghi, O. M. Metal-organic frameworks: A new class of porous materials. Microporous Mesoporous Mater. 2004 , 73 , 3–14.
Deng, H. X.; Grunder, S.; Cordova, K. E.; Valente, C.; Furukawa, H.; Hmadeh, M.; Gándara, F.; Whalley, A. C.; Liu, Z.; Asahina, S. et al. Large-pore apertures in a series of metal-organic frameworks. Science 2012 , 336 , 1018–1023.
Ojha, R. P.; Lemieux, P. A.; Dixon, P. K.; Liu, A. J.; Durian, D. J. Statistical mechanics of a gas-fluidized particle. Nature 2004 , 427 , 521–523.
Férey, G.; Mellot-Draznieks, C.; Serre, C.; Millange, F.; Dutour, J.; Surblé, S.; Margiolaki, I. A chromium terephthalate-based solid with unusually large pore volumes and surface area. Science 2005 , 309 , 2040–2042.
Kandiah, M.; Nilsen, M. H.; Usseglio, S.; Jakobsen, S.; Olsbye, U.; Tilset, M.; Larabi, C.; Quadrelli, E. A.; Bonino, F.; Lillerud, K. P. Synthesis and stability of tagged UiO-66 Zr-MOFs. Chem. Mater. 2010 , 22 , 6632–6640.
Zou, L. F.; Feng, D. W.; Liu, T. F.; Chen, Y. P.; Yuan, S.; Wang, K. C.; Wang, X.; Fordham, S.; Zhou, H. C. A versatile synthetic route for the preparation of titanium metal-organic frameworks. Chem. Sci. 2016 , 7 , 1063–1069.
Andreo, J.; Priola, E.; Alberto, G.; Benzi, P.; Marabello, D.; Proserpio, D. M.; Lamberti, C.; Diana, E. Autoluminescent metal-organic frameworks (MOFs): Self-photoemission of a highly stable thorium MOF. J. Am. Chem. Soc. 2018 , 140 , 14144–14149.
Sanii, R.; Hua, C.; Patyk-Kaźmierczak, E.; Zaworotko, M. J. Solvent-directed control over the topology of entanglement in square lattice (sql) coordination networks. Chem. Commun. 2019 , 55 , 1454–1457.
Xie, L. S.; Alexandrov, E. V.; Skorupskii, G.; Proserpio, D. M.; Dincă, M. Diverse π-π stacking motifs modulate electrical conductivity in tetrathiafulvalene-based metal-organic frameworks. Chem. Sci. 2019 , 10 , 8558–8565.
Muldoon, P. F.; Liu, C.; Miller, C. C.; Koby, S. B.; Jarvi, A. G.; Luo, T. Y.; Saxena, S.; O’Keeffe, M.; Rosi, N. L. Programmable topology in new families of heterobimetallic metal-organic frameworks. J. Am. Chem. Soc. 2018 , 140 , 6194–6198.
Fischer, S.; Roeser, J.; Lin, T. C.; DeBlock, R. H.; Lau, J.; Dunn, B. S.; Hoffmann, F.; Frçba, M.; Thomas, A.; Tolbert, S. H. A metal-organic framework with tetrahedral aluminate sites as a single-ion Li + solid electrolyte. Angew. Chem., Int. Ed. 2018 , 57 , 16683–16687.
Rubio-Martinez, M.; Avci-Camur, C.; Thornton, A. W.; Imaz, I.; Maspoch, D.; Hill, M. R. New synthetic routes towards MOF production at scale. Chem. Soc. Rev. 2017 , 46 , 3453–3480.
Lidström, P.; Tierney, J.; Wathey, B.; Westman J. Microwave assisted organic synthesis: A review. Tetrahedron 2001 , 57 , 9225–9283.
Jhung, S. H.; Lee, J. H.; Chang, J. S. Microwave synthesis of a nanoporius hybrid material, chromium trimesate. Bull. Korean Chem. Soc. 2005 , 26 , 880–881.
Choi, J. S.; Son, W. J.; Kim, J.; Ahn, W. S. Metal-organic framework MOF-5 prepared by microwave heating: Factors to be considered. Microporous Mesoporous Mater. 2008 , 116 , 727–731.
Son, W. J.; Kim, J.; Kim, J.; Ahn, W. S. Sonochemical synthesis of MOF-5. Chem. Commun. 2008 , 6336–6338.
Li, M. Y.; Dincă, M. Reductive electrosynthesis of crystalline metal-organic frameworks. J. Am. Chem. Soc. 2011 , 133 , 12926–12929.
Chen, Y. F.; Li, S. Q.; Pei, X. K.; Zhou, J. W.; Feng, X.; Zhang, S. H.; Cheng, Y. Y.; Li, H. W.; Han, R. D.; Wang, B. A solvent-free hot-pressing method for preparing metal-organic-framework coatings. Angew. Chem., Int. Ed. 2016 , 55 , 3419–3423.
McKinstry, C.; Cathcart, R. J.; Cussen, E. J.; Fletcher, A. J.; Patwardhan, S. V.; Sefcik, J. Scalable continuous solvothermal synthesis of metal organic framework (MOF-5) crystals. Chem. Eng. J. 2016 , 285 , 718–725.
Seo, Y. K.; Hundal, G.; Jang, I. T.; Hwang, Y. K.; Jun, C. H.; Chang, J. S. Microwave synthesis of hybrid inorganic-organic materials including porous Cu 3 (BTC) 2 from Cu(II)-trimesate mixture. Microporous Mesoporous Mater. 2009 , 119 , 331–337.
Li, Z. Q.; Qiu, L. G.; Xu, T.; Wu, Y.; Wang, W.; Wu, Z. Y.; Jiang, X. Ultrasonic synthesis of the microporous metal-organic framework Cu 3 (BTC) 2 at ambient temperature and pressure: An efficient and environmentally friendly method. Mater. Lett. 2009 , 63 , 78–80.
Nawrocki, J.; Prochowicz, D.; Wiśniewski, A.; Justyniak, I.; Goś, P.; Lewiński, J. Development of an SBU-based mechanochemical approach for drug-loaded MOFs. Eur. J. Inorg. Chem. 2020 , 2020 , 796–800.
Crawford, D.; Casaban, J.; Haydon, R.; Giri, N.; McNally, T.; James, S. L. Synthesis by extrusion: Continuous, large-scale preparation of MOFs using little or no solvent. Chem. Sci. 2015 , 6 , 1645–1649.
Carné-Sánchez, A.; Imaz, I.; Cano-Sarabia, M.; Maspoch, D. A spray-drying strategy for synthesis of nanoscale metal-organic frameworks and their assembly into hollow superstructures. Nat. Chem. 2013 , 5 , 203–211.
Ameloot, R.; Vermoortele, F.; Vanhove, W.; Roeffaers, M. B. J.; Sels, B. F.; De Vos, D. E. Interfacial synthesis of hollow metal-organic framework capsules demonstrating selective permeability. Nat. Chem. 2011 , 3 , 382–387.
Yang, D. A.; Cho, H. Y.; Kim, J.; Yang, S. T.; Ahn, W. S. CO 2 capture and conversion using Mg-MOF-74 prepared by a sonochemical method. Energy Environ. Sci. 2012 , 5 , 6465–6473.
Wu, X. F.; Bao, Z. B.; Yuan, B.; Wang, J.; Sun, Y. Q.; Luo, H. M.; Deng, S. G. Microwave synthesis and characterization of MOF-74 (M = Ni, Mg) for gas separation. Microporous Mesoporous Mater. 2013 , 180 , 114–122.
Ayoub, G.; Karadeniz, B.; Howarth, A. J.; Farha, O. K.; Ðilović, I.; Germann, L. S.; Dinnebier, R. E.; Užarević, K.; Friščić, T. Rational synthesis of mixed-metal microporous metal-organic frameworks with controlled composition using mechanochemistry. Chem. Mater. 2019 , 31 , 5494–5501.
Cavka, J. H.; Jakobsen, S.; Olsbye, U.; Guillou, N.; Lamberti, C.; Bordiga, S.; Lillerud, K. P. A New zirconium inorganic building brick forming metal organic frameworks with exceptional stability. J Am. Chem. Soc. 2008 , 130 , 13850–13851.
Karadeniz, B.; Howarth, A. J.; Stolar, T.; Islamoglu, T.; Dejanović, I.; Tireli, M.; Wasson, M. C.; Moon, S. Y.; Farha, O. K.; Friščić, T. et al. Benign by design: Green and scalable synthesis of zirconium UiO-metal-organic frameworks by water-assisted mechanochemistry. ACS Sustainable Chem. Eng. 2018 , 6 , 15841–15849.
Avci-Camur, C.; Troyano, J.; Pérez-Carvajal, J.; Legrand, A.; Farrusseng, D.; Imaz, I.; Maspoch, D. Aqueous production of spherical Zr-MOF beads via continuous-flow spray-drying. Green Chem. 2018 , 20 , 873–878.
Taddei, M.; Steitz, D. A.; Van Bokhoven, J. A.; Ranocchiari, M. Continuous-flow microwave synthesis of metal-organic frameworks: A highly efficient method for large-scale production. Chem. —Eur. J. 2016 , 22 , 3245–3249.
Park, K. S.; Ni, Z.; Côté, A. P.; Choi, J. Y.; Huang, R. D.; Uribe-Romo, F. J.; Chae, H. K.; O’Keeffe, M.; Yaghi, O. M. Exceptional chemical and thermal stability of zeolitic imidazolate frameworks. Proc. Natl. Acad. Sci. USA 2006 , 103 , 10186–10191.
Chaemchuen, S.; Zhou, K.; Mousavi, B.; Ghadamyari, M.; Heynderickx, P. M.; Zhuiykov, S.; Yusubov, M. S.; Verpoort, F. Spray drying of zeolitic imidazolate frameworks: Investigation of crystal formation and properties. CrystEngComm 2018 , 20 , 3601–3608.
Polyzoidis, A.; Altenburg, T.; Schwarzer, M.; Loebbecke, S.; Kaskel, S. Continuous microreactor synthesis of ZIF-8 with high space-time-yield and tunable particle size. Chem. Eng. J. 2016 , 283 , 971–977.
Laybourn, A.; Katrib, J.; Ferrari-John, R. S.; Morris, C. G.; Yang, S. H.; Udoudo, O.; Easun, T. L.; Dodds, C.; Champness, N. R.; Kingman, S. W. et al. Metal-organic frameworks in seconds via selective microwave heating. J. Mater. Chem A 2017 , 5 , 7333–7338.
Ni, Z.; Masel, R. I. Rapid production of metal-organic frameworks via microwave-assisted solvothermal synthesis. J. Am. Chem. Soc. 2006 , 128 , 12394–12395.
Liu, B. T.; He, Y. P.; Han, L. P.; Singh, V.; Xu, X. N.; Guo, T.; Meng, F. Y.; Xu, X.; York, P.; Liu, Z. X. et al. Microwave-assisted rapid synthesis of γ-cyclodextrin metal-organic frameworks for size control and efficient drug loading. Cryst. Growth Des. 2017 , 17 , 1654–1660.
Jiang, Z. W.; Zou, Y. C.; Zhao, T. T.; Zhen, S. J.; Li, Y. F.; Huang, C. Z. Controllable synthesis of porphyrin-based 2D lanthanide metal-organic frameworks with thickness- and metal-node-dependent photocatalytic performance. Angew. Chem., Int. Ed. 2020 , 59 , 3300–3306.
Haque, E.; Khan, N. A.; Park, J. H.; Jhung, S. H. Synthesis of a metal-organic framework material, iron terephthalate, by ultrasound, microwave, and conventional electric heating: A kinetic study. Chem. —Eur. J. 2010 , 16 , 1046–1052.
Dreischarf, A. C.; Lammert, M.; Stock, N.; Reinsch, H. Green synthesis of Zr-CAU-28: Structure and properties of the first Zr-MOF based on 2, 5-furandicarboxylic acid. Inorg. Chem. 2017 , 56 , 2270–2277.
Jacobsen, J.; Achenbach, B.; Reinsch, H.; Smolders, S.; Lange, F. D.; Friedrichs, G.; De Vos, D.; Stock, N. The first water-based synthesis of Ce(IV)-MOFs with saturated chiral and achiral C 4 -dicarboxylate linkers. Dalton Trans. 2019 , 48 , 8433–8441.
Lee, Y. R.; Kim, J.; Ahn, W. S. Synthesis of metal-organic frameworks: A mini review. Korean J. Chem. Eng. 2013 , 30 , 1667–1680.
Suslick, K. S.; Hammerton, D. A.; Cline, R. E. Jr. Sonochemical hot spot. J. Am. Chem. Soc. 1986 , 108 , 5641–5642.
Qiu, L. G.; Li, Z. Q.; Wu, Y.; Wang, W.; Xu, T.; Jiang, X. Facile synthesis of nanocrystals of a microporous metal-organic framework by an ultrasonic method and selective sensing of organoamines. Chem. Commun. 2008 , 3642–3644.
Vaitsis, C.; Sourkouni, G.; Argirusis, C. Metal organic frameworks (MOFs) and ultrasound: A review. Ultrason. Sonochem. 2019 , 52 , 106–119.
Zhao, S. L.; Wang, Y.; Dong, J. C.; He, C. T.; Yin, H. J.; An, P. F.; Zhao, K.; Zhang, X. F.; Gao, C.; Zhang, L. J. et al. Ultrathin metal-organic framework nanosheets for electrocatalytic oxygen evolution. Nat. Energy 2016 , 1 , 16184.
Li, C.; Hu, X. S.; Tong, W.; Yan, W. S.; Lou, X. B.; Shen, M.; Hu, B. W. Ultrathin manganese-based metal-organic framework nanosheets: Low-cost and energy-dense lithium storage anodes with the coexistence of metal and ligand redox activities. ACS Appl. Mater. Interfaces 2017 , 9 , 29829–29838.
Ning, Y. Q.; Lou, X. B.; Li, C.; Hu, X. S.; Hu, B. W. Ultrathin cobalt-based metal-organic framework nanosheets with both metal and ligand redox activities for superior lithium storage. Chem. —Eur. J. 2017 , 23 , 15984–15990.
Yuan, M. W.; Wang, R.; Fu, W. B.; Lin, L.; Sun, Z. M.; Long, X. G.; Zhang, S. T.; Nan, C. Y.; Sun, G. B.; Li, H. F. et al. Ultrathin two-dimensional metal-organic framework nanosheets with the inherent open active sites as electrocatalysts in aprotic Li-O 2 batteries. ACS Appl. Mater. Interfaces 2019 , 11 , 11403–11413.
Kim, J.; Yang, S. T.; Choi, S. B.; Sim, J.; Kim, J.; Ahn, W. S. Control of catenation in CuTATB -n metal-organic frameworks by sonochemical synthesis and its effect on CO 2 adsorption. J. Mater. Chem. 2011 , 21 , 3070–3076.
Li, J. X.; Li, Y. H.; Qin, Z. B.; Dong, G. Y. Ultrasound assisted synthesis of a zinc(II) coordination polymer with nano-flower morphology and the use as precursor for zinc(II) oxide nanoparticles. Polyhedron 2018 , 155 , 94–101.
Mueller, U.; Puetter, H.; Hesse, M.; Wessel, H.W. Method for electrochemical production of a crystalline porous metal organic skeleton material. WO 2005/049892, February 6, 2005.
Wei, J. Z.; Wang, X. L.; Sun, X. J.; Hou, Y.; Zhang, X.; Yang, D. D.; Dong, H.; Zhang, F. M. Rapid and large-scale synthesis of IRMOF-3 by electrochemistry method with enhanced fluorescence detection performance for TNP. Inorg. Chem. 2018 , 57 , 3818–3824.
Wei, J. Z.; Gong, F. X.; Sun, X. J.; Li, Y.; Zhang, T.; Zhao, X. J.; Zhang, F. M. Rapid and low-cost electrochemical synthesis of UiO-66-NH 2 with enhanced fluorescence detection performance. Inorg. Chem. 2019 , 58 , 6742–6747.
Kang, X. C.; Lyu, K.; Li, L. L.; Li, J. N.; Kimberley, L.; Wang, B.; Liu, L. F.; Cheng, Y. Q.; Frogley, M. D.; Rudić, S. et al. Integration of mesopores and crystal defects in metal-organic frameworks via templated electrosynthesis. Nat. Commun. 2019 , 10 , 4466.
Li, W. J.; Tu, M.; Cao, R.; Fischer, R. A. Metal-organic framework thin films: Electrochemical fabrication techniques and corresponding applications & perspectives. J. Mater. Chem. A 2016 , 4 , 12356–12369.
Li, W. J.; Lü, J.; Gao, S. Y.; Li, Q. H.; Cao, R. Electrochemical preparation of metal-organic framework films for fast detection of nitro explosives. J. Mater. Chem. A 2014 , 2 , 19473–19478.
Li, M. Y.; Dincă, M. Selective formation of biphasic thin films of metal-organic frameworks by potential-controlled cathodic electrodeposition. Chem. Sci. 2014 , 5 , 107–111.
Liu, Y. X.; Wei, Y. N.; Liu, M. H.; Bai, Y. C.; Wang, X. Y.; Shang, S. C.; Chen, J. Y.; Liu, Y. Q. Electrochemical synthesis of large area two-dimensional metal-organic framework films on copper anodes. Angew. Chem., Int. Ed. 2021 , 60 , 2887–2891.
Zhou, S.; Shekhah, O.; Jia, J. T.; Czaban-Jóźwiak, J.; Bhatt, P. M.; Ramírez, A.; Gascon, J.; Eddaoudi, M. Electrochemical synthesis of continuous metal-organic framework membranes for separation of hydrocarbons. Nat. Energy 2021 , 6 , 882–891.
Pichon, A.; Lazuen-Garay, A.; James, S. L. Solvent-free synthesis of a microporous metal-organic framework. CrystEngComm 2006 , 8 , 211–214.
Li, R.; Ren, X. Q.; Zhao, J. S.; Feng, X.; Jiang, X.; Fan, X. X.; Lin, Z. G.; Li, X. G.; Hu, C. W.; Wang, B. Polyoxometallates trapped in a zeolitic imidazolate framework leading to high uptake and selectivity of bioactive molecules. J. Mater. Chem. A 2014 , 2 , 2168–2173.
Fidelli, A. M.; Karadeniz, B.; Howarth, A. J.; Huskić, I.; Germann, L. S.; Halasz, I.; Etter, M.; Moon, S. Y.; Dinnebier, R. E.; Stilinović, V. et al. Green and rapid mechanosynthesis of high-porosity NU- and UiO-type metal-organic frameworks. Chem. Commun. 2018 , 54 , 6999–7002.
Karadeniz, B.; Žilić, D.; Huskić, I.; Germann, L. S.; Fidelli, A. M.; Muratović, S.; Lončarić, I.; Etter, M.; Dinnebier, R. E.; Barišić, D. et al. Controlling the polymorphism and topology transformation in porphyrinic zirconium metal-organic frameworks via mechanochemistry. J. Am. Chem. Soc. 2019 , 141 , 19214–19220.
Lee, H. K.; Lee, J. H.; Moon, H. R. Mechanochemistry as a reconstruction tool of decomposed metal-organic frameworks. Inorg. Chem. 2021 , 60 , 11825–11829.
Crawford, D. E.; Casaban, J. Recent developments in mechanochemical materials synthesis by extrusion. Adv. Mater. 2016 , 28 , 5747–5754.
Darwish, S.; Wang, S. Q.; Croker, D. M.; Walker, G. M.; Zaworotko, M. J. Comparison of mechanochemistry vs solution methods for synthesis of 4,4’-bipyridine-based coordination polymers. ACS Sustainable Chem. Eng. 2019 , 7 , 19505–19512.
Abramova, A.; Couzon, N.; Leloire, M.; Nerisson, P.; Cantrel, L.; Royer, S.; Loiseau, T.; Volkringer, C.; Dhainaut, J. Extrusion-spheronization of UiO-66 and UiO-66_NH 2 into robust-shaped solids and their use for gaseous molecular iodine, xenon, and krypton adsorption. ACS Appl. Mater. Interfaces 2022 , 14 , 10669–10680.
Quan, Y. F.; Shen, R. Q.; Ma, R.; Zhang, Z. R.; Wang, Q. S. Sustainable and efficient manufacturing of metal-organic framework-based polymer nanocomposites by reactive extrusion. ACS Sustainable Chem. Eng. 2022 , 10 , 7216–7222.
Ma, X. J.; Chai, Y. T.; Li, P.; Wang, B. Metal-organic framework films and their potential applications in environmental pollution control. Acc. Chem. Res. 2019 , 1461–1470.
Chen, Y. F.; Zhang, S. H.; Cao, S. J.; Li, S. Q.; Chen, F.; Yuan, S.; Xu, C.; Zhou, J. W.; Feng, X.; Ma, X. J. et al. Roll-to-roll production of metal-organic framework coatings for particulate matter removal. Adv. Mater. 2017 , 29 , 1606221.
Tang, Y. J.; Chen, Y. F.; Zhu, H. J.; Zhang, A. M.; Wang, X. L.; Dong, L. Z.; Li, S. L.; Xu, Q.; Lan, Y. Q. Solid-phase hot-pressing synthesis of POMOFs on carbon cloth and derived phosphides for all pH value hydrogen evolution. J. Mater. Chem. A 2018 , 6 , 21969–21977.
Li, G. P.; Cao, F.; Zhang, K.; Hou, L.; Gao, R. C.; Zhang, W. Y.; Wang, Y. Y. Design of anti-UV radiation textiles with self-assembled metal-organic framework coating. Adv. Mater. Interfaces 2020 , 7 , 1901525.
Li, P.; Li, J. Z.; Feng, X.; Li, J.; Hao, Y. C.; Zhang, J. W.; Wang, H.; Yin, A. X.; Zhou, J. W.; Ma, X. J. et al. Metal-organic frameworks with photocatalytic bactericidal activity for integrated air cleaning. Nat. Commun. 2019 , 10 , 2177.
Wang, H.; Zhao, S.; Liu, Y.; Yao, R. X.; Wang, X. Q.; Cao, Y. H.; Ma, D.; Zou, M. C.; Cao, A. Y.; Feng, X. et al. Membrane adsorbers with ultrahigh metal-organic framework loading for high flux separations. Nat. Commun. 2019 , 10 , 4204.
Gauvin, W. H.; Katta, S. Basic concepts of spray dryer design. AIChE J. 1976 , 22 , 713–724.
Garzón-Tovar, L.; Cano-Sarabia, M.; Carné-Sánchez, A.; Carbonell, C.; Imaz, I.; Maspoch, D. A spray-drying continuous-flow method for simultaneous synthesis and shaping of microspherical high nuclearity MOF beads. React. Chem. Eng. 2016 , 1 , 533–539.
Avci, C.; De Marco, M. L.; Byun, C.; Perrin, J.; Scheel, M.; Boissière, C.; Faustini, M. Metal-organic framework photonic balls: Single object analysis for local thermal probing. Adv. Mater. 2021 , 33 , 2104450.
Sun, J. Z.; Kwon, H. T.; Jeong, H. K. Continuous synthesis of high quality metal-organic framework HKUST-1 crystals and composites via aerosol-assisted synthesis. Polyhedron 2018 , 153 , 226–233.
Stassen, I.; Styles, M.; Grenci, G.; Van Gorp, H.; Vanderlinden, W.; De Feyter, S.; Falcaro, P.; De Vos, D.; Vereecken, P.; Ameloot, R. Chemical vapour deposition of zeolitic imidazolate framework thin films. Nat. Mater. 2016 , 15 , 304–310.
Britton, J.; Jamison, T. F. The assembly and use of continuous flow systems for chemical synthesis. Nat. Protoc. 2017 , 12 , 2423–2446.
Albuquerque, G. H.; Fitzmorris, R. C.; Ahmadi, M.; Wannenmacher, N.; Thallapally, P. K.; McGrail, B. P.; Herman, G. S. Gas-liquid segmented flow microwave-assisted synthesis of MOF-74(Ni) under moderate pressures. CrystEngComm 2015 , 17 , 5502–5510.
Liu, X. M.; Xie, L. H.; Wu, Y. F. Recent advances in the shaping of metal-organic frameworks. Inorg. Chem. Front. 2020 , 7 , 2840–2866.
Download references
Acknowledgements
This work was financially supported by the National Natural Science Foundation of China (Nos. 21971017, 21901019, and 22205018), the National Key Research and Development Program of China (No. 2020YFB1506300), and Beijing Institute of Technology Research Fund Program. The authors acknowledge the Analysis and Testing Center of BIT for technical supports.
Author information
Authors and affiliations.
Frontiers Science Center for High Energy Material, Beijing Key Laboratory of Photoelectronic/Electrophotonic Conversion Materials, Key Laboratory of Cluster Science, School of Chemistry and Chemical Engineering, Beijing Institute of Technology, Beijing, 100081, China
Dou Ma, Xin Huang, Lu Wang & Bo Wang
Advanced Technology Research Institute (Jinan), Beijing Institute of Technology, Ji’nan, 250000, China
Dou Ma & Bo Wang
Beijing Institute of Technology Library, Beijing Institute of Technology, Beijing, 100081, China
You can also search for this author in PubMed Google Scholar
Corresponding authors
Correspondence to Lu Wang or Bo Wang .
Rights and permissions
Reprints and permissions
About this article
Ma, D., Huang, X., Zhang, Y. et al. Metal-organic frameworks: Synthetic methods for industrial production. Nano Res. 16 , 7906–7925 (2023). https://doi.org/10.1007/s12274-023-5441-4
Download citation
Received : 06 October 2022
Revised : 29 November 2022
Accepted : 27 December 2022
Published : 10 March 2023
Issue Date : May 2023
DOI : https://doi.org/10.1007/s12274-023-5441-4
Share this article
Anyone you share the following link with will be able to read this content:
Sorry, a shareable link is not currently available for this article.
Provided by the Springer Nature SharedIt content-sharing initiative
- metal-organic frameworks
- synthetic methods
- crystallization mechanisms
- Find a journal
- Publish with us
- Track your research
Thank you for visiting nature.com. You are using a browser version with limited support for CSS. To obtain the best experience, we recommend you use a more up to date browser (or turn off compatibility mode in Internet Explorer). In the meantime, to ensure continued support, we are displaying the site without styles and JavaScript.
- View all journals
- My Account Login
- Explore content
- About the journal
- Publish with us
- Sign up for alerts
- Open access
- Published: 26 April 2024
Local gate control of Mott metal-insulator transition in a 2D metal-organic framework
- Benjamin Lowe ORCID: orcid.org/0000-0002-5157-7737 1 , 2 na1 ,
- Bernard Field ORCID: orcid.org/0000-0003-0352-9156 1 , 2 na1 ,
- Jack Hellerstedt ORCID: orcid.org/0000-0003-2282-8223 1 , 2 ,
- Julian Ceddia ORCID: orcid.org/0000-0003-3990-8852 1 , 2 ,
- Henry L. Nourse 3 ,
- Ben J. Powell ORCID: orcid.org/0000-0002-5161-1317 4 ,
- Nikhil V. Medhekar ORCID: orcid.org/0000-0003-3124-4430 2 , 5 &
- Agustin Schiffrin ORCID: orcid.org/0000-0003-1140-8485 1 , 2
Nature Communications volume 15 , Article number: 3559 ( 2024 ) Cite this article
3 Altmetric
Metrics details
- Electronic properties and materials
- Molecular electronics
- Phase transitions and critical phenomena
Electron-electron interactions in materials lead to exotic many-body quantum phenomena, including Mott metal-insulator transitions (MITs), magnetism, quantum spin liquids, and superconductivity. These phases depend on electronic band occupation and can be controlled via the chemical potential. Flat bands in two-dimensional (2D) and layered materials with a kagome lattice enhance electronic correlations. Although theoretically predicted, correlated-electron Mott insulating phases in monolayer 2D metal-organic frameworks (MOFs) with a kagome structure have not yet been realised experimentally. Here, we synthesise a 2D kagome MOF on a 2D insulator. Scanning tunnelling microscopy (STM) and spectroscopy reveal a MOF electronic energy gap of ∼ 200 meV, consistent with dynamical mean-field theory predictions of a Mott insulator. Combining template-induced (via work function variations of the substrate) and STM probe-induced gating, we locally tune the electron population of the MOF kagome bands and induce Mott MITs. These findings enable technologies based on electrostatic control of many-body quantum phases in 2D MOFs.
Similar content being viewed by others
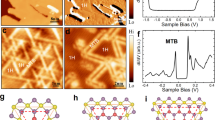
Electronic Janus lattice and kagome-like bands in coloring-triangular MoTe2 monolayers
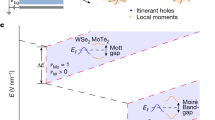
Gate-tunable heavy fermions in a moiré Kondo lattice
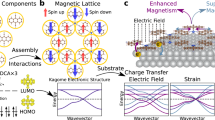
Correlation-induced magnetism in substrate-supported 2D metal-organic frameworks
Introduction.
Strong electronic correlations arise in a material at specific electron fillings of its bands, provided that the on-site Coulomb repulsion (characterised by the Hubbard energy, U ) is of the order of, or larger than, the bandwidth, W . These electronic correlations can result in a wide range of exotic many-body quantum phases. Examples include correlated insulating phases, quantum spin liquids, correlated magnetism, and superconductivity—phenomena that have been realised in monolayer transition metal-dichalcogenides 1 , 2 , 3 , 4 , 5 , 6 , twisted few-layer graphene 7 , 8 , inorganic kagome crystals 9 , 10 , 11 , and organic charge transfer salts 12 , 13 , 14 .
Tuning of the chemical potential via electrostatic gating can allow for control over such band electron filling, enabling reversible switching between correlated phases 7 . This makes these systems amenable to integration as active materials in voltage-controlled devices, offering enticing prospects for applications in electronics, spintronics, and information processing and storage 12 , 15 .
Two-dimensional (2D) materials have emerged as particularly promising candidates for realising strongly correlated phenomena as the absence of interlayer hopping and screening can contribute to decreasing W and increasing U 4 . Additionally, some 2D crystal geometries—such as the kagome structure—give rise to intrinsic flat electronic bands 16 , 17 . When these extremely narrow bands are half-filled, even weak Coulomb repulsion can open an energy gap and give rise to a Mott insulating phase 12 . Away from half-filling, the gap closes, and the system becomes metallic.
Metal-organic frameworks (MOFs) are a broad class of materials whose properties are highly tunable through careful selection of constituent organic molecules and metal atoms 18 . There has been growing interest in 2D MOFs for their electronic properties 19 , 20 , 21 . In particular, layered 2D MOF structures have been recently shown to host strongly correlated superconductivity 22 . Monolayer 2D MOFs have attracted attention for their magnetism 23 , 24 , 25 , including ferromagnetism resulting from exchange interaction between unpaired metal centre electrons 26 . However, despite theoretical predictions 27 , 28 , correlated Mott phases have not yet been realised experimentally in monolayer 2D MOFs.
Here, we demonstrate local electrostatic control over a Mott metal-insulator-transition (MIT) in a single-layer 2D kagome MOF, in excellent agreement with theoretical predictions.
A monolayer 2D MOF on an atomically thin insulator
We synthesised the monolayer MOF—consisting of 9,10-dicyanoanthracene (DCA) molecules coordinated to copper (Cu) atoms—on monolayer hexagonal boron nitride (hBN) on Cu(111) (see Methods for sample preparation). A scanning tunnelling microscopy (STM) image of a crystalline single-layer MOF domain grown seamlessly across the hBN/Cu(111) substrate is shown in Fig. 1 a. We observe some defects within the MOF domain, as well as some DCA-only regions (discussed in Supplementary Note 11) . The long-range modulation of the MOF STM apparent height follows the hBN/Cu(111) moiré pattern, which arises due to a mismatch between the hBN and Cu(111) lattices (giving rise to pore, P, and wire, W, regions—see upper inset) 29 , 30 , 31 . This moiré pattern has been shown to affect the electronic properties of adsorbates 31 , including one previous example of a MOF 32 .
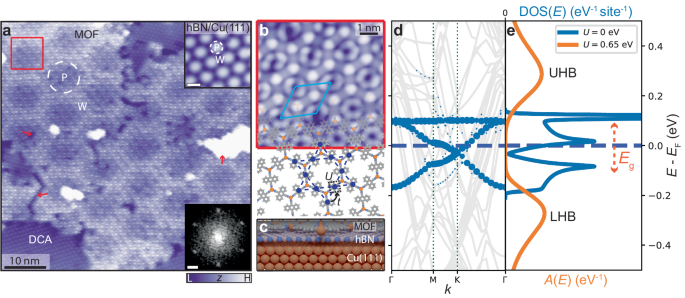
a STM image of MOF (with organic DCA-only regions; V b = −1 V, I t = 10 pA). 'P' and 'W' indicate pore (dashed white circle) and wire regions of hBN/Cu(111) moiré pattern. Lower inset: Fourier transform of STM image; sharp spots correspond to MOF hexagonal periodicity (scale bar: 0.25 nm −1 ). Upper inset: STM image of bare hBN/Cu(111) moiré pattern ( V b = 4 V, I t = 100 pA, scale bar: 4 nm). Red arrows: examples of crack, vacancy, and Cu cluster defects within MOF domain. b Top-view of MOF model overlaid upon small-scale STM image of region within red box in a ( V b = −1 V, I t = 10 pA). MOF unit cell indicated in light blue. Blue dashed lines and solid circles: kagome pattern formed by DCA molecules, with inter-site electron hopping, t , and on-site Coulomb repulsion, U . c Model of MOF/hBN/Cu(111) (side view). Hydrogen: white; carbon: grey; boron: pink; nitrogen: blue; copper: orange. d Electronic band structure calculated by DFT (with U = 0) 36 . Blue circles: projections onto MOF states. Grey curves: Cu(111) states (hBN states do not contribute within the shown energy range). Hybridisation between MOF and Cu(111) is hindered by the hBN monolayer; the MOF band structure retains its kagome character. Chemical potential E F (blue dashed line) is close to half-filling of kagome bands. e Density of states, DOS( E ) (tight-binding model with thermal broadening, U = 0, blue), and spectral function, A ( E ) (DMFT, U = 0.65 eV, orange), of freestanding MOF. Local Coulomb interaction opens a significant Mott energy gap E g between lower (LHB) and upper Hubbard bands (UHB).
The MOF is characterised by a hexagonal lattice (lattice constant: 2.01 ± 0.06 nm), with a unit cell including two Cu atoms (honeycomb arrangement; bright protrusions in Fig. 1 b) and three DCA molecules (kagome arrangement, with protrusions at both ends of anthracene backbone in STM image in Fig. 1 b), similar to previous reports (including on a decoupling graphene monolayer) 25 , 33 , 34 , 35 .
We calculated the band structure of this monolayer DCA 3 Cu 2 MOF on hBN/Cu(111) by density functional theory (DFT; with U = 0); Fig. 1 d. Projection of the Kohn–Sham wavefunctions onto MOF states shows the prototypical kagome energy dispersion with two Dirac bands and a flat band, consistent with prior theoretical calculations for the freestanding MOF 19 , 27 , 36 . This near-Fermi band structure has predominantly molecular DCA character, and is well described by a nearest-neighbour tight-binding (TB) model (see the corresponding density of electronic states, DOS, as a function of energy E in Fig. 1 e) 36 . The hBN monolayer, a 2D insulator with a bandgap >5 eV 31 , prevents electronic hybridisation between the underlying Cu(111) surface and the 2D MOF 31 . This allows the MOF to preserve its intrinsic electronic properties, in contrast to previous findings on metal surfaces 25 , 35 , 36 , 37 . These U = 0 calculations predict that the MOF on hBN/Cu(111) is metallic, with some electron transfer from substrate-to-MOF leading to the chemical potential lying above the Dirac point, close to half-filling of the three kagome bands (Fig. 1 d).
Strong electronic interactions have been theoretically predicted in DCA 3 Cu 2 27 , and a signature was recently detected experimentally 25 . We, therefore, calculated the many-body spectral function A ( E )—analogous to the DOS in the non-interacting regime—of the freestanding MOF via dynamical mean-field theory (DMFT). In contrast to the TB model or DFT, DMFT explicitly captures local electronic correlations caused by the Hubbard energy U (see Methods) 38 , 39 , 40 , 41 . In Fig. 1 e, A ( E ) is shown for U = 0.65 eV (consistent with previous experimental estimates 33 ) and for a chemical potential that matches the DFT-predicted occupation of the kagome bands for the MOF on hBN/Cu(111) (see Supplementary Note 2 and Supplementary Note 23 for further DMFT calculations, including temperature dependence). We observe two broad peaks (lower and upper Hubbard bands) separated by an energy gap of ∼ 200 meV, dramatically different from the non-interacting kagome DOS, and indicative of a Mott insulating phase 27 , 42 .
Observation of ∼ 200 meV Mott energy gap
To experimentally probe the electronic properties of DCA 3 Cu 2 /hBN/Cu(111), we conducted differential conductance (d I /d V ) scanning tunnelling spectroscopy (STS); d I /d V is an approximation of the local DOS [ A ( E )] in the non-interacting (interacting, respectively) picture. We performed STS at the ends of the DCA anthracene moiety and at the Cu sites of the MOF—locations where we expect the strongest signature of the kagome bands based on the spatial distribution of the orbitals that give rise to these bands 25 , 33 , 34 . These spectra (Fig. 2 a), taken at a pore region of the hBN/Cu(111) moiré pattern, both show broad peaks at bias voltages V b ≈ −0.2 and 0.2 V. In a bias voltage window of ∼ 0.2 V around the chemical potential E F ( V b = 0), the d I /d V signal is low, significantly smaller than that for bare hBN/Cu(111).
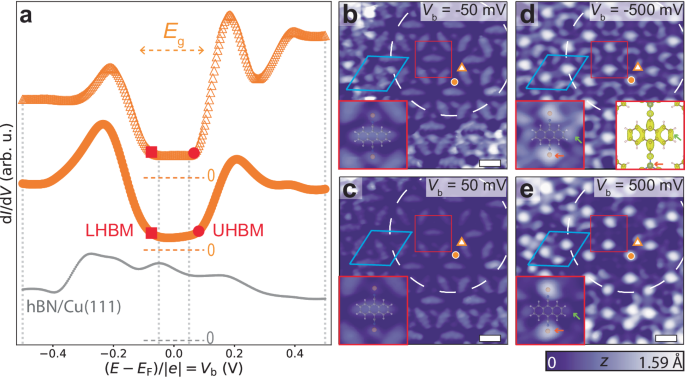
a d I /d V spectra at MOF positions indicated by orange markers in b – e and on bare hBN/Cu(111) (grey dots) (setpoint: V b = −500 mV, I t = 500 pA). Grey dotted vertical lines indicate bias voltages at which STM images were acquired in b – e . Spectra offset for clarity. Dashed horizontal lines indicate d I /d V = 0 reference for each curve. Spectra reveal a bandgap E g ≈ 200 meV. The non-zero d I /d V within the gap is due to states of underlying Cu(111) leaking through hBN. Red squares (circles): lower Hubbard band maxima, LHBM (upper Hubbard band minima, UHBM). b – e STM images of MOF on hBN/Cu(111) at specified bias voltages ( I t = 10 pA). White dashed circle: hBN/Cu(111) moiré pore. MOF unit cell indicated in light blue. Scale bars: 1 nm. Insets: zoom-in of region within red box, with overlaid Cu-DCA-Cu chemical structure, showing significant contributions from the ends of the DCA anthracene moiety (green arrow) and Cu (orange arrow) for V b < LHBM and V b > UHBM. Right inset in d : charge density isosurface (0.0025 e − Å −3 ) of DCA 3 Cu 2 obtained by integration of near-Fermi (±0.5 eV) DFT wavefunctions.
STM images acquired within this low-d I /d V bias voltage window (Fig. 2 b, c) show mainly the topography of the MOF, with the molecules appearing as ellipses of uniform intensity and the Cu atoms as weak protrusions. Outside the low-d I /d V bias voltage window ( ∣ V b ∣ > 200 mV), Cu sites and the ends of the DCA anthracene moieties appear as bright protrusions (Fig. 2 d, e), similar to the spatial distribution of the electronic orbitals of the DCA 3 Cu 2 MOF associated with the near-Fermi kagome bands (right inset of Fig. 2 d; see Supplementary Figs. 11 and 12 for more V b -dependent STM images and d I /d V maps) 25 , 33 , 34 .
This suggests that the d I /d V peaks at ∣ V b ∣ ≈ 0.2 V in Fig. 2 a are related to intrinsic MOF electronic states near E F , with the low-d I /d V bias voltage window of ∼ 0.2 V around E F representing an energy gap, E g , between these states. This is consistent with in-gap topographic STM imaging in Fig. 2 b, c 43 . These d I /d V peaks cannot be attributed to inelastic tunnelling (e.g., MOF vibrational modes) as they are not always symmetric about E F (see Fig. 3 ). The gap is much larger than that predicted from spin-orbit coupling in such a system 19 , and the monocrystalline growth of the MOF domains (Fig. 1 a) makes a large disorder-related gap unlikely. Furthermore, the gap is inconsistent with DFT and TB calculations (Fig. 1 d, e).
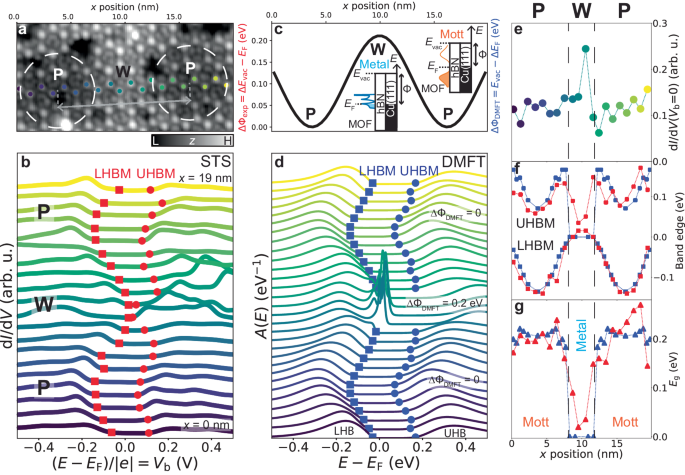
a STM image of MOF ( V b = −1 V, I t = 10 pA). White dashed circles (P): hBN/Cu(111) moiré pores, separated by wire (W). Grey arrow indicates moiré period λ ≈ 12.5 nm. b d I /d V spectra acquired at MOF Cu sites, at positions indicated by coloured markers in a (tip 190 pm further from STM setpoint V b = 10 mV, I t = 10 pA). Energy gap E g ≈ 200 meV at P regions, vanishing at W region (LHBM: lower Hubbard band maximum; UHBM: upper Hubbard band minimum). c Sinusoidal variation of work function, ΔΦ = Δ E vac − E F ( E vac : vacuum energy level), across hBN/Cu(111) moiré domain with periodicity λ ≈ 12.5 nm 30 , affecting the MOF electron filling. d Spectral functions A ( E ) calculated via DMFT ( U = 0.65 eV, t = 0.05 eV) for isolated uniform DCA 3 Cu 2 , for different values of E F . We account for experimental corrugation ΔΦ by varying E F sinusoidally with an amplitude of 0.2 eV as per c (see Methods). e Experimental d I /d V signal at Fermi level ( V b = 0) as a function of x position, from b . Increased d I /d V ( V b = 0) indicates metallic phase. f , g Experimental (red; b ) and DMFT (blue; d ) LHBM (squares), UHBM (circles), and energy gap E g (triangles), as a function of x in a (experiment) or corresponding E F (DMFT).
The MOF spectra in Fig. 2 a strongly resemble the DMFT-calculated spectral function A ( E ) in Fig. 1 e, including an energy gap of the same magnitude between two similar peaks (Supplementary Fig. 9) . This suggests that these d I /d V spectra are hallmarks of a Mott insulator.
Template-assisted Mott metal-insulator transition (MIT)
We further measured d I /d V spectra at Cu sites of the DCA 3 Cu 2 MOF across the hBN/Cu(111) moiré pattern (Fig. 3 a, b). In Fig. 3 b, E g is centred symmetrically about E F for spectra taken in the middle of a pore region, while those taken closer to the wire region show the Hubbard bands shifting upwards in energy (lowering the barrier to creation of a hole). At the centre of the wire region, the gap at the Fermi level vanishes with a clear increase in Fermi level d I /d V signal (Fig. 3 e). The same behaviour was observed for DCA lobe sites of the MOF (see Supplementary Note 14) .
The hBN/Cu(111) moiré pattern consists of a modulation of the local work function Φ (with little structural corrugation), where the quantity \(\Delta \Phi={\Phi }_{{{{{{{{\rm{wire}}}}}}}}}-{\Phi }_{{{{{{{{\rm{pore}}}}}}}}}\) depends on the period of the moiré superstructure, λ 29 , 30 , 31 . For the hBN/Cu(111) domain in Fig. 3 a with λ ≈ 12.5 nm, ΔΦ ≈ 0.2 eV (Fig. 3 c; see Supplementary Note 15 for moiré domains with different periods) 30 . Due to energy level alignment 44 , 45 , 46 , this corrugation of Φ affects substrate-to-MOF electron transfer and hence the effective electron filling of the MOF bands, with this filling smaller at wire than pore regions 44 , 47 . This is consistent with the effective reduction of the hole-creation barrier at the wire relative to the pore in Fig. 3 b.
To capture the effect of this moiré-induced modulation of Φ on the MOF electronic properties, we conducted further DMFT calculations. Using U = 0.65 eV (the same as Fig. 1 e), we calculated A ( E ) for a range of E F assuming a uniform system. We considered a sinusoidal variation of E F from a minimum value corresponding to half-filling of the kagome MOF bands, with an amplitude of 0.2 eV, to match the experimental ΔΦ for this specific hBN/Cu(111) moiré domain 30 , 47 (Fig. 3 c; see Methods). The obtained A ( E ) (Fig. 3 d) reproduce the experimental spectral features in Fig. 3 b, including the shifting of the lower Hubbard band maximum (LHBM) and upper Hubbard band minimum (UHBM) (Fig. 3 f), and the vanishing of the gap and increase of the spectral function at the Fermi level for the wire region (Fig. 3 e, g).
The DMFT-calculated spectral functions A ( E ) with no gap at the Fermi level at the smallest electron filling (ΔΦ DMFT = 0.2 eV) show peaks near E F (Fig. 3 d), however, which were not observed in the experimental spectra at the wire region (Fig. 3 b). These peaks are indicative of coherent quasiparticles 38 , with their width associated with the quasiparticle lifetime and quasiparticle mean free path ℓ . Via our DMFT and TB calculations (Supplementary Note 3) , we estimate ℓ ≈ 10 nm, much larger than the wire region width of ∼ 4 nm. We hypothesise that the coherence peaks are suppressed in the experiment as quasiparticles are strongly scattered by the pore regions (where the MOF remains insulating). This is a key difference between Fig. 3 b, d: the experimental measurements represent changes in electron population at finite size MOF regions due to a locally varying work function, whereas each theoretical spectrum corresponds to an infinite uniform system with a constant chemical potential.
Furthermore, some of the experimental spectra in the vicinity of the wire region in Fig. 3 b feature narrow peaks at energies of ∼ 0.4 eV, not present in the theoretical A ( E ) in Fig. 3 d. We claim that these peaks do not represent intrinsic electronic states, but are instead related to charging phenomena not captured by the DMFT calculations (see Fig. 4 and Supplementary Note 17) .
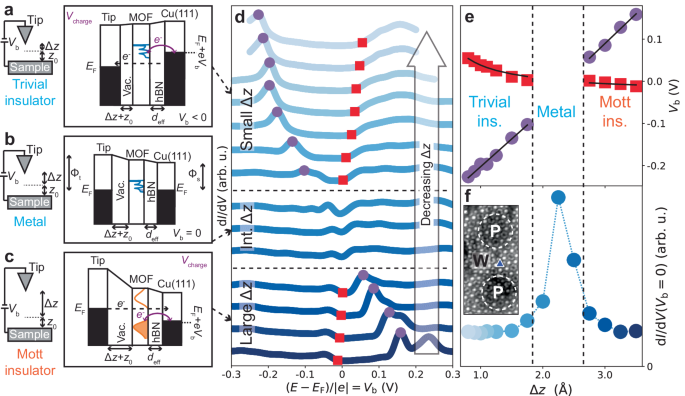
a – c Schematics and energy diagrams of tunnelling and charging processes at a double-barrier tunnel junction (DBTJ), consisting of STM tip, vacuum barrier (vac.), MOF, hBN barrier, and Cu(111), for small, intermediate (int.), and large tip-sample distances (Δ z + z 0 ). When V b is applied, voltage drop at MOF location enables tip-controlled charging, energy level shifts, and gating of MOF transitions, from (correlated) Mott insulator to metal to trivial insulator. These schematics qualitatively illustrate our proposed physical mechanism of tip-induced gating. d d I /d V spectra at MOF DCA lobe site, at hBN/Cu(111) moiré wire, for different Δ z + z 0 ( z 0 given by STM setpoint V b = 10 mV, I t = 10 pA). Purple circles: MOF charging peak. Red squares: intrinsic electronic state at MOF band edge. Spectra normalised and offset for clarity. e V charge (purple circles in d ) and V state (red squares in d ) as a function of Δ z . Black solid lines: global fits to Eqs. ( 1 ) and ( 2 ). f d I /d V signal at Fermi level ( V b = 0) as a function of Δ z , from d . Increased d I /d V ( V b = 0) indicates metallic phase at intermediate Δ z + z 0 . Inset: STM image of MOF on hBN/Cu(111) showing site (blue triangle marker) where d I /d V (Δ z ) measurements were performed ( V b = − 1 V, I t = 10 pA, scale bar: 2 nm).
Despite these discrepancies with experiment, our DMFT calculations capture the fundamental electronic properties of the 2D DCA 3 Cu 2 MOF, hosting a Mott insulating phase with E g ≈ 200 meV, and a metal-like phase (with no gap at the Fermi level) at the wire region (for the specific tip-sample distance considered in Fig. 3) . DMFT is a well-established method for understanding the Mott insulator and Mott MITs 38 , 39 , 40 , 41 . This claim of strong electronic correlations and of a Mott insulating phase for the DCA 3 Cu 2 kagome MOF is consistent with previous literature 25 , 27 , 36 , 42 .
Tip-assisted Mott MIT at moiré wire region
To explore the nature of the metal-like phase observed at the wire region, we conducted further d I /d V STS of the MOF as a function of tip-sample distance Δ z + z 0 (where z 0 is set by tunnelling parameters), at a DCA lobe site within the wire region (Fig. 4 d). For large Δ z + z 0 , these spectra feature an energy gap E g , with a small d I /d V signal at the Fermi level (Fig. 4 f), similar to spectra in the pore regions (Figs. 2 a, 3 b; Supplementary Note 20) , with a sharp peak at positive V b (purple circles in Fig. 4 d) and a subtler band edge (red squares; similar to band features in Fig. 3 b) at negative V b . As Δ z decreases, the energy position of the sharp peak decreases linearly (Fig. 4 e). Conversely, the energy position of the subtler band edge increases with decreasing Δ z , non-linearly and at a lower rate (Fig. 4 e). These features cross the Fermi level at intermediate Δ z , and the spectrum becomes gapless ( E g = 0), with a significant increase in d I /d V signal at the Fermi level (Fig. 4 f). Note that an intermediate Δ z was also used for all spectra in Fig. 3 b where similar metal-like signatures were observed at the wire region. We found a similar Δ z -dependent trend for wire region Cu sites (Supplementary Note 21) . Notably, moiré pore regions remain gapped for all Δ z values (for both Cu and DCA lobe sites; Supplementary Note 20 and Supplementary Note 21) .
As V b is applied between the tip and Cu substrate, the STM double-barrier tunnel junction (DBTJ)—where the vacuum between tip and MOF is a first tunnel barrier and the insulating hBN is a second one—causes a voltage drop at the MOF location. This can lead to energy shifts of MOF states and/or charging of such states when they become resonant with the Cu(111) Fermi level (Fig. 4 a) 33 , 46 , 48 . These phenomena have previously been observed for the DCA 3 Cu 2 MOF on a decoupling graphene surface 33 . In this scenario, the bias voltages corresponding to an intrinsic electronic state, V state , and to charging of such a state, V charge , vary as a function of Δ z as:
where d eff is the effective width of the hBN tunnel barrier, V ∞ is the bias voltage corresponding to the electronic state as Δ z → ∞ , and ΔΦ ts is the difference between tip and sample work functions 48 .
We fit the Δ z -dependent bias voltage associated with the subtle band edge (red squares) in Fig. 4 d with Eq. ( 1 ), and the bias voltage associated with the sharp peak (purple circles) with Eq. ( 2 ) (Fig. 4 e). The agreement between experimental data and fits indicate that the subtle spectral band edge (red) represents an intrinsic MOF electronic state, with its energy shifting as Δ z varies, and with the sharp peak (purple) corresponding to charging of such a state.
We interpret these results as follows. For large Δ z (Fig. 4 c), the MOF electronic states are strongly pinned to the substrate, and the MOF near-Fermi kagome bands are approximately half-filled. Here, the d I /d V spectra feature an energy gap at the Fermi level, both at moiré pore (Figs. 2 a, 3 b; Supplementary Note 20 and Supplementary Note 21) and wire regions (bottom spectra of Fig. 4 d; Supplementary Note 21) : the entire monolayer 2D MOF is intrinsically a Mott insulator featuring localised electrons. This is consistent with DMFT calculations, which show that the system can remain Mott insulating even with a 0.2 eV modulation of E F (corresponding to the experimental moiré work function corrugation; Supplementary Fig. 4) .
Due to the hBN/Cu(111) moiré work function corrugation, the LHBM at a wire region is very close to the Fermi level (in comparison to the pore region). As Δ z is reduced, the MOF states become less pinned to the Cu(111) substrate and more pinned to the tip via the DBTJ effect (Fig. 4 ; Supplementary Note 19 , Supplementary Note 20 , Supplementary Note 21) . Given that \({\Phi }_{{{{{{{{\rm{tip}}}}}}}}} \, > \, {\Phi }_{{{{{{{{\rm{wire}}}}}}}}}\) (see Supplementary Note 18) , this leads to an energy upshift of the MOF states with respect to the Cu(111) Fermi level (with the LHBM susceptible to charging as V b becomes more positive). At intermediate Δ z , this energy upshift depopulates the LHB and leads to the transition from the Mott insulating phase (only existing at half-filling of the three near-Fermi kagome bands; Fig. 1) to the metallic phase. This is concomitant with a dramatic change in the d I /d V spectra, including the vanishing of the energy gap and the increase in d I /d V signal at the Fermi level. As Δ z is further reduced (Fig. 4 a), the near-Fermi kagome bands become fully depopulated (with the bottom of these bands susceptible to charging as V b becomes more negative; top spectra of Fig. 4 d), and the MOF becomes a trivial insulator (with a gap between these near-Fermi bands and lower energy bands; Supplementary Fig. 1) . The DBTJ effect also manifests itself at the pore region, with energy shifts of the LHBM and UHBM as Δ z varies (Supplementary Note 20 , Supplementary Note 21) . Yet, due to the smaller \({\Phi }_{{{{{{{{\rm{pore}}}}}}}}}\) (Fig. 3 c), the Fermi level lies close to the centre of the energy gap (Fig. 3 b), making the Mott insulating phase robust for the considered Δ z range, consistent with DMFT (Fig. 3 d, Supplementary Figs. 2 b, 4) . In the wire region, the STM tip, via the DBTJ effect, in combination with the large \({\Phi }_{{{{{{{{\rm{wire}}}}}}}}}\) , acts as a local electrostatic gate, switching the 2D MOF from Mott insulator to metal (Supplementary Fig. 26) .
This tip-induced gating at the wire region is inherently local, occurring within the cross-section of the DBTJ (typically with a diameter of ∼ 10 nm given by the tip radius of curvature). This locality could lead to d I /d V features (e.g., due to electronic confinement) not captured by DMFT. Whether these local changes produce truly delocalised metallic states across an extended area of the sample remains an open question, beyond the scope of this investigation. Future work could investigate the DCA 3 Cu 2 MOF on single-crystal exfoliated hBN, within a gated heterostructure, allowing for uniform electrostatic control and bulk (e.g., transport) measurements.
Our assertion that the MOF is a Mott insulator at the moiré pore regions for all tip-sample distances, and at the wire regions for large tip-sample distances, is well supported by DMFT calculations, and by previous literature 25 , 27 , 36 , 42 . The DMFT spectral functions demonstrate excellent agreement with d I /d V spectra at the pore regions, including the ∼ 200 meV gap at the Fermi level, and the energy modulation of the LHB and UHB due to variations in electrostatic potential. Our assertion of a metallic phase for the MOF at the wire region for intermediate tip-sample distances is well supported by the DBTJ model, and by the qualitative agreement with DMFT, including the absence of an energy gap at the Fermi level and an increase in Fermi level d I /d V signal and spectral function.
Mott insulating—with intrinsically localised electronic states—and metallic phases have been observed in TaS 2 and TaSe 2 monolayers, which feature frustrated lattice geometries and lattice constants (due to charge density wave distortions) similar to our work 3 , 4 , 49 , 50 , 51 , for finite domain sizes as small as ~10 × 10 nm 2 49 . Also, local topological phase transitions induced by an STM tip have been demonstrated 52 . Our interpretation of a local population-induced Mott metal-insulator transition is consistent with these findings.
Monolayer DCA-based MOFs have been studied on other substrates 25 , 26 , 33 , 34 , 35 , 37 , 53 , 54 , 55 , without observing a Mott phase. In our case, the combination of the wide bandgap hBN as a template (allowing the MOF to retain its intrinsic electronic properties), and of the adequate energy level alignment given by the hBN/Cu(111) substrate (resulting in half-filling of kagome bands; Fig. 1 d), plays a key role in the realisation of the correlated-electron Mott phase.
Note that the vanishing of the energy gap at the Fermi level for the MOF metallic phase at the moiré wire region (Fig. 3b ) can be reminiscent of spectral features for the pseudogap phase in cuprates and other transition metal oxides 56 , 57 , 58 . Future work on controllable Mott MITs in MOFs might shed light on the general understanding of doped Mott insulators.
We have demonstrated that single-layer DCA 3 Cu 2 not only hosts a robust Mott insulating phase (with E g ≫ k B T at T = 300 K), but also that Mott MITs can be achieved via the combination of template- (Fig. 3) and tip- (Fig. 4) induced gating, consistent with DMFT and the DBTJ model. This shows that such phase transitions can be controlled in monolayer MOFs via electrostatic tuning of the chemical potential.
Our findings represent a promising step towards incorporation of 2D MOFs as active materials in device-like architectures (e.g., van der Waals heterostructures based on 2D materials), benefiting from efficient synthesis approaches and versatility offered by MOFs, and allowing for access and control of correlated-electron phases therein via electrostatic gating 59 . Our work establishes single-layer 2D MOFs—with crystal geometries allowing for flat bands—as promising platforms for controllable switching between diverse many-body quantum phenomena, potentially including correlated magnetism, superconductivity, and quantum spin liquids.
Sample preparation
The monolayer (ML) DCA 3 Cu 2 kagome MOF was synthesised on hBN/Cu(111) in UHV (base pressure ∼ 2 × 10 −10 mbar). The Cu(111) surface was first cleaned via 2–3 cycles of sputtering with Ar + ions and subsequent annealing at ∼ 770 K. A hBN ML was synthesised on Cu(111) via the thermal decomposition of borazine 31 . We dosed a partial pressure of borazine of ∼ 9 × 10 −7 mbar for 45 minutes with the Cu(111) sample maintained at 1140 K. We kept the Cu(111) sample at this temperature for a further 20 mins to ensure a complete reaction. We then cooled the sample to room temperature and deposited the DCA molecules via sublimation at 390 K, corresponding to a deposition rate of 0.007 ML/sec. In our experiments we considered DCA coverages of ∼ 0.4–0.6 ML. We then further cooled the sample to ∼ 77 K before depositing Cu via sublimation at 1250 K (Cu deposition rate: ∼ 0.002 ML/sec; typical Cu coverages in our experiments: ∼ 0.05 ML). Finally, the sample was annealed to ∼ 200 K for 15 minutes. Further details are in Supplementary Note 6 .
The DCA 3 Cu 2 MOF crystalline structure was found to be commensurate with the hBN lattice but incommensurate with the long-range hBN/Cu(111) moiré patterns of different sizes, across which the MOF grows without disruption (see Supplementary Fig. 8) . The hBN/Cu(111) moiré pattern is clearly visible in large-scale STM images of the MOF (Fig. 1 a). This is consistent with the modulation of the MOF’s electronic properties illustrated in Fig. 3 (also see d I /d V maps in Supplementary Fig. 12) .
STM and STS measurements
All STM and d I /d V STS measurements were performed at 4.5 K (except measurements in Supplementary Note 23 performed at 77 K), at a base pressure <1 × 10 −10 mbar, with a hand-cut Pt/Ir tip. All STM images were acquired in constant-current mode with tunnelling parameters as reported in the text (bias voltage applied to sample). All d I /d V spectra were obtained by acquiring I ( V ) at a constant tip-sample distance (stabilised by a specified setpoint tunnelling current and bias voltage), and by then numerically differentiating I ( V ) to obtain d I /d V as a function of bias voltage. Tips were characterised on regions of bare hBN/Cu(111) prior to spectroscopy measurements, where the Shockley surface state of Cu(111) could be observed (grey curve in Fig. 2 a, onset shifted upwards in energy due to confinement by hBN monolayer) 29 .
DFT calculations
We calculated the non-spin-polarised band structure of DCA 3 Cu 2 on hBN on Cu(111) via DFT (Fig. 1 d), using the Vienna Ab-Initio Simulation Package 60 with the Perdew-Burke-Ernzerhof functional under the generalised gradient approximation 61 . We used projector augmented wave pseudopotentials 62 , 63 to describe core electrons, and the semi-empirical potential DFT-D3 64 to describe van der Waals forces.
The substrate was modelled as a slab three Cu atoms thick, with the bottom layer fixed at the bulk lattice constant 65 . A layer of passivating hydrogen atoms was applied to the bottom face to terminate dangling bonds.
A 400 eV cut-off was used for the plane wave basis set. The threshold for energy convergence was 10 −4 eV. The atomic positions of the DCA 3 Cu 2 /hBN/Cu(111) were relaxed until Hellmann-Feynman forces were <0.01 eV/Å, using a 3 × 3 × 1 k-point grid for sampling the Brillouin zone and 1st order Methfessel-Paxton smearing of 0.2 eV. The charge density for the relaxed structure was calculated using an 11 × 11 × 1 k-point grid, Blöchl tetrahedron interpolation, and dipole corrections. The band structure was determined non-self-consistently from the charge density.
Note that small (<1 Å) perturbations in the height of DCA 3 Cu 2 above the hBN/Cu(111) do not appreciably affect the calculated band structure. As such, small perturbations in height related to the hBN/Cu(111) moiré pattern (of at most 0.7 Å 66 ) were not captured by these calculations 36 .
DMFT calculations
We performed DMFT calculations on the freestanding DCA 3 Cu 2 kagome MOF. We used the Hubbard model for a kagome lattice with nearest-neighbour hopping,
where the first term is the TB Hamiltonian with nearest-neighbour hopping energy t , ∑ 〈 i , j 〉 is a sum over nearest-neighbour sites, and the second term is the interaction Hamiltonian with on-site Coulomb repulsion U . The operator \({\hat{c}}_{i,\sigma }^{{{{\dagger}}} }\) ( \({\hat{c}}_{i,\sigma }\) ) creates (annihilates) an electron at site i with spin σ ; \({\hat{n}}_{i,\sigma }={\hat{c}}_{i,\sigma }^{{{{\dagger}}} }{\hat{c}}_{i,\sigma }\) is the density operator. We take t = 0.05 eV to match prior DFT calculations of DCA 3 Cu 2 19 , 27 , 36 .
We first calculated the non-interacting ( U = 0) density of states (DOS; blue curve in Fig. 1 e) by numerically integrating over all momenta in the first Brillouin zone. The chemical potential E F in Fig. 1 e was chosen to be consistent with the electron filling predicted by DFT (Fig. 1 d). We applied a thermal broadening ( k B T = 2.5 meV) to this non-interacting TB DOS to make it consistent with the thermal broadening of the DMFT-generated (see below) spectral function A ( E ) in Fig. 1 e.
To account for electronic correlations, we then implemented the DMFT formalism 38 , 67 , 68 using the Toolbox for Researching Interacting Quantum Systems (TRIQS) 69 , with the continuous-time hybridisation expansion solver (CTHYB) 70 , 71 at a temperature of ∼ 29 K ( k B T ≈ 0.05 t, unless specified otherwise; see Supplementary Note 23 for temperature-dependent calculations), with U = 0.65 eV. To use a single-site DMFT formalism 38 with the kagome band structure, the non-interacting DOS of the three kagome bands were combined into a single function for use as the input into the DMFT procedure.
We calculated the many-body spectral functions A ( E ) (analogous to the DOS, but in the interacting regime; Fig. 3 d) via analytic continuation using the maximum entropy method (MaxEnt) as implemented 72 in TRIQS. The meta-parameter, α , was determined from the maximum curvature of the distance between the MaxEnt fit and data, χ 2 , as a function of α 73 .
Each DMFT calculation assumed a spatially uniform work function Φ; long-range modulation of Φ is beyond the capabilities of DMFT. As such, the spatially varying sample work function Φ resulting from the experimental hBN/Cu(111) moiré pattern was not explicitly captured in the individual A ( E ) spectra in Fig. 3 d. This spatial variation of Φ was approximated by varying the uniform E F of the system for each individual A ( E ) spectrum. Each of these A ( E ) spectra was then associated to a specific location of the hBN/Cu(111) moiré pattern (and hence to a specific experimental d I /d V curve) based on how this variation of E F would translate to a local Φ. These calculations assume that the theoretical A ( E ) spectra, calculated with a uniform E F (and hence uniform Φ), are reasonable representations of the locally acquired experimental d I /d V curves, which are affected by a spatially varying Φ. This assumption is reasonable for the insulating phase. Indeed, in the Mott insulating phase, electronic states are localised at the kagome sites, confined within areas that are small in length 74 compared to the distance between nearest-neighbour kagome sites ( ∼ 1 nm) and to the periodicity λ of the hBN/Cu(111) moiré domains considered in our experiments ( λ > 5 nm). DMFT indicates that this Mott insulating phase is robust to variations in chemical potential E F larger than 0.2 eV, with the spectral function A ( E ) shifting in energy as E F is varied within this range, without other significant qualitative changes (see Supplementary Fig. 2 b for E F = 0.25 to 0.5 eV). In our experiments, the hBN/Cu(111) moiré pattern imposes a periodic modulation of the local work function Φ, with a peak-to-peak modulation amplitude of ∼ 0.2 eV and a modulation periodicity λ ≈ 12.5 nm (Fig. 3 c; this amplitude becomes smaller with decreasing λ , see Supplementary Fig. 22) . That is, Φ varies slowly across the MOF kagome lattice. The effect of such long-range modulation of Φ is to shift the energy of the MOF localised states accordingly. As long as the Φ modulation period is larger than the distance between nearest-neighbour kagome sites and the Φ modulation amplitude is smaller than a critical value inducing the transition to the metallic phase, there is no other dramatic qualitative effect on these localised electronic states. This explains the excellent agreement between experimental d I /d V spectra for the MOF at the hBN/Cu(111) moiré pore region and DMFT-calculated spectral functions A ( E ) for the system in the Mott insulating phase (Fig. 3 b, d), with LHB and UHB modulated in energy following the variation in electrostatic potential. For the MOF in the metallic phase at the moiré wire region (Figs. 3 , 4) , discrepancies between theory and experiment can be explained (as discussed in the main text) by long electronic coherence lengths (Supplementary Note 3) , and by effects of long-range moiré Φ modulation and of finite DBTJ cross section on the potentially delocalised metallic MOF states.
In Fig. 4 d, e, the bias voltage, V state , corresponds to the energy level of an intrinsic MOF frontier electronic state (which is susceptible to charging), at a band edge in either the Mott or trivial insulator regime. Red square markers indicating V state were assigned based on the method outlined in Supplementary Note 13 . The bias voltage, V charge , corresponds to the peak associated with charging of such a MOF state. Purple circle markers indicating V charge were assigned by finding a local maximum in d I /d V .
In Fig. 4 e, we considered four experimental datasets for fitting with Eqs. ( 1 ) and ( 2 ): V state (Δ z ) (red squares) and V charge (Δ z ) (purple circles) for small values of Δ z (trivial insulator phase), and V state (Δ z ) and V charge (Δ z ) for large values of Δ z (Mott insulator phase). Given the Mott MIT, we considered two different intrinsic MOF band edges susceptible to charging, embodied in two different values of V ∞ : one for the trivial insulator phase (small values of Δ z ) and one for the Mott insulator phase (large Δ z ). This phase transition is evident from the offset in V state (red squares in Fig. 4 e) observed when Δ z varies from small to large (through the metallic phase at intermediate Δ z ). Accordingly, we used a global fitting approach to obtain the same fitting parameters d eff , z 0 , and ΔΦ ts (characteristic of the DBTJ and the acquisition location) for these four experimental datasets, and a separate V ∞ value for each regime.
Schematics in Fig. 4 a–c represent cartoon illustrations of our proposed physical mechanism for tip-induced gating.
It is important to note that the DBTJ inherently affects all d I /d V measurements in this work, including those in Figs. 2 and 3 , for both pore and wire regions of the hBN/Cu(111) moiré pattern. The DBTJ effect does not cause phase transitions at the pore regions, however (see Supplementary Note 20 , Supplementary Note 21) . The measurements in Fig. 3 were performed with an intermediate tip-sample distance—which is why metallic properties were observed at the wire region.
Data availability
The data supporting the findings of this study is available from the authors upon request.
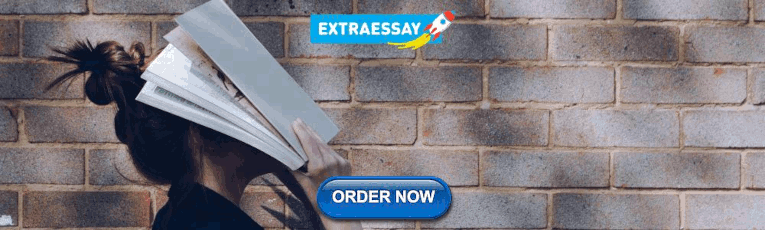
Code availability
All codes relating to DMFT and MaxEnt are available at https://doi.org/10.5281/zenodo.7439858 . The authors can provide code for data analysis and theoretical calculations upon request.
Nakata, Y. et al. Monolayer 1T-NbSe 2 as a Mott insulator. NPG Asia Mater. 8 , e321 (2016).
Article CAS Google Scholar
Liu, M. et al. Monolayer 1T-NbSe 2 as a 2D-correlated magnetic insulator. Sci. Adv. 7 , eabi6339 (2021).
Article ADS CAS PubMed Google Scholar
Lin, H. et al. Scanning tunneling spectroscopic study of monolayer 1T-TaS 2 and 1T-TaSe 2 . Nano Res. 13 , 133–137 (2020).
Chen, Y. et al. Strong correlations and orbital texture in single-layer 1T-TaSe 2 . Nat. Phys. 16 , 218–224 (2020).
Ruan, W. et al. Evidence for quantum spin liquid behaviour in single-layer 1T-TaSe 2 from scanning tunnelling microscopy. Nat. Phys. 17 , 1154–1161 (2021).
Chen, Y. et al. Evidence for a spinon Kondo effect in cobalt atoms on single-layer 1T-TaSe 2 . Nat. Phys. 18 , 1335–1340 (2022).
Cao, Y. et al. Unconventional superconductivity in magic-angle graphene superlattices. Nature 556 , 43–50 (2018).
Park, J. M., Cao, Y., Watanabe, K., Taniguchi, T. & Jarillo-Herrero, P. Tunable strongly coupled superconductivity in magic-angle twisted trilayer graphene. Nature 590 , 249–255 (2021).
Zheng, L. et al. Emergent charge order in pressurized kagome superconductor CsV 3 Sb 5 . Nature 611 , 682–687 (2022).
Ye, L. et al. Massive Dirac fermions in a ferromagnetic kagome metal. Nature 555 , 638–642 (2018).
Yin, J.-X. et al. Quantum-limit Chern topological magnetism in TbMn 6 Sn 6 . Nature 583 , 533–536 (2020).
Powell, B. J. & McKenzie, R. H. Quantum frustration in organic Mott insulators: from spin liquids to unconventional superconductors. Rep. Prog. Phys. 74 , 056501 (2011).
Article ADS Google Scholar
Kanoda, K. & Kato, R. Mott physics in organic conductors with triangular lattices. Annu. Rev. Condens. Matter Phys. 2 , 167–188 (2011).
Article ADS CAS Google Scholar
Miksch, B. et al. Gapped magnetic ground state in quantum spin liquid candidate κ -(BEDT-TTF) 2 Cu 2 (CN) 3 . Science 372 , 276–279 (2021).
Shao, Z., Cao, X., Luo, H. & Jin, P. Recent progress in the phase-transition mechanism and modulation of vanadium dioxide materials. NPG Asia Mater. 10 , 581–605 (2018).
Article Google Scholar
Balents, L., Dean, C. R., Efetov, D. K. & Young, A. F. Superconductivity and strong correlations in moiré flat bands. Nat. Phys. 16 , 725–733 (2020).
Neupert, T., Denner, M. M., Yin, J.-X., Thomale, R. & Hasan, M. Z. Charge order and superconductivity in kagome materials. Nat. Phys. 18 , 137–143 (2022).
Cui, Y. et al. Metal-organic frameworks as platforms for functional materials. Acc. Chem. Res. 49 , 483–493 (2016).
Article CAS PubMed Google Scholar
Zhang, L. Z. et al. Intrinsic two-dimensional organic topological insulators in metal-dicyanoanthracene lattices. Nano Lett. 16 , 2072–2075 (2016).
Jin, Y. et al. Large-gap quantum anomalous Hall phase in hexagonal organometallic frameworks. Phys. Rev. B 98 , 245127 (2018).
Jiang, W., Ni, X. & Liu, F. Exotic topological bands and quantum states in metal-organic and covalent-organic frameworks. Acc. Chem. Res. 54 , 416–426 (2021).
Takenaka, T. et al. Strongly correlated superconductivity in a copper-based metal-organic framework with a perfect kagome lattice. Sci. Adv. 7 , eabf3996 (2021).
Yamada, M. G., Fujita, H. & Oshikawa, M. Designing Kitaev spin liquids in metal-organic frameworks. Phys. Rev. Lett. 119 , 057202 (2017).
Article ADS PubMed Google Scholar
Zhang, L.-C. et al. Two-dimensional magnetic metal-organic frameworks with the Shastry-Sutherland lattice. Chem. Sci. 10 , 10381–10387 (2019).
Kumar, D. et al. Manifestation of strongly correlated electrons in a 2D kagome metal-organic framework. Adv. Funct. Mater. 31 , 2106474 (2021).
Lobo-Checa, J. et al. Ferromagnetism on an atom-thick & extended 2D metal-organic coordination network. Nat. Commun. 15 , 1858 (2024).
Fuchs, M. et al. Kagome metal-organic frameworks as a platform for strongly correlated electrons. J. Phys. Mater. 3 , 025001 (2020).
Nourse, H. L., McKenzie, R. H. & Powell, B. J. Multiple insulating states due to the interplay of strong correlations and lattice geometry in a single-orbital Hubbard model. Phys. Rev. B 103 , L081114 (2021).
Joshi, S. et al. Boron nitride on Cu(111): an electronically corrugated monolayer. Nano Lett. 12 , 5821–5828 (2012).
Zhang, Q. et al. Tuning band gap and work function modulations in monolayer hBN/Cu(111) heterostructures with moiré patterns. ACS Nano 12 , 9355–9362 (2018).
Auwärter, W. Hexagonal boron nitride monolayers on metal supports: versatile templates for atoms, molecules and nanostructures. Surf. Sci. Rep. 74 , 1–95 (2019).
Urgel, J. I. et al. Controlling coordination reactions and assembly on a Cu(111) supported boron nitride monolayer. J. Am. Chem. Soc. 137 , 2420–2423 (2015).
Yan, L. et al. Synthesis and local probe gating of a monolayer metal-organic framework. Adv. Funct. Mater. 31 , 2100519 (2021).
Yan, L. et al. Two-dimensional metal-organic framework on superconducting NbSe 2 . ACS Nano 15 , 17813–17819 (2021).
Hernández-López, L. et al. Searching for kagome multi-bands and edge states in a predicted organic topological insulator. Nanoscale 13 , 5216–5223 (2021).
Article PubMed Google Scholar
Field, B., Schiffrin, A. & Medhekar, N. V. Correlation-induced magnetism in substrate-supported 2D metal-organic frameworks. npj Comput Mater. 8 , 1–10 (2022).
Pawin, G. et al. A surface coordination network based on substrate-derived metal adatoms with local charge excess. Angew. Chem. Int. Ed. 47 , 8442–8445 (2008).
Georges, A., Kotliar, G., Krauth, W. & Rozenberg, M. J. Dynamical mean-field theory of strongly correlated fermion systems and the limit of infinite dimensions. Rev. Mod. Phys. 68 , 13–125 (1996).
Article ADS MathSciNet CAS Google Scholar
Kotliar, G. & Vollhardt, D. Strongly correlated materials: insights from dynamical mean-field theory. Phys. Today 57 , 53–59 (2004).
Vollhardt, D. Dynamical mean-field theory for correlated electrons. Ann. Phys. 524 , 1–19 (2012).
Adler, R., Kang, C.-J., Yee, C.-H. & Kotliar, G. Correlated materials design: prospects and challenges. Rep. Prog. Phys. 82 , 012504 (2018).
Ohashi, T., Kawakami, N. & Tsunetsugu, H. Mott transition in kagome lattice hubbard model. Phys. Rev. Lett. 97 , 066401 (2006).
Repp, J., Meyer, G., Stojković, S. M., Gourdon, A. & Joachim, C. Molecules on insulating films: scanning-tunneling microscopy imaging of individual molecular orbitals. Phys. Rev. Lett. 94 , 026803 (2005).
Joshi, S. et al. Control of molecular organization and energy level alignment by an electronically nanopatterned boron nitride template. ACS Nano 8 , 430–442 (2014).
Zimmermann, D. M. et al. Self-assembly and spectroscopic fingerprints of photoactive pyrenyl tectons on hBN/Cu(111). Beilstein J. Nanotechnol. 11 , 1470–1483 (2020).
Pörtner, M. et al. Charge state control of F 16 CoPc on h-BN/Cu(111). Adv. Mater. Interfaces 7 , 2000080 (2020).
Kumar, D., Hellerstedt, J., Lowe, B. & Schiffrin, A. Mesoscopic 2D molecular self-assembly on an insulator. Nanotechnology 34 , 205601 (2023).
ADS Google Scholar
Kumar, D., Krull, C., Yin, Y., Medhekar, N. V. & Schiffrin, A. Electric field control of molecular charge state in a single-component 2D organic nanoarray. ACS Nano 13 , 11882–11890 (2019).
Vaňo, V. et al. Artificial heavy fermions in a van der Waals heterostructure. Nature 599 , 582–586 (2021).
Bu, K. et al. Possible strain induced Mott gap collapse in 1T-TaS2. Commun. Phys. 2 , 1–7 (2019).
Fei, Y., Wu, Z., Zhang, W. & Yin, Y. Understanding the Mott insulating state in 1T-TaS2 and 1T-TaSe2. AAPPS Bull. 32 , 20 (2022).
Collins, J. L. et al. Electric-field-tuned topological phase transition in ultrathin Na3Bi. Nature 564 , 390–394 (2018).
Kumar, A., Banerjee, K., Foster, A. S. & Liljeroth, P. Two-dimensional band structure in honeycomb metal-organic frameworks. Nano Lett. 18 , 5596–5602 (2018).
Yan, L., Pohjavirta, I., Alldritt, B. & Liljeroth, P. On-surface assembly of Au-dicyanoanthracene coordination structures on Au(111). ChemPhysChem 20 , 2297–2300 (2019).
Vaňo, V. et al. Emergence of exotic spin texture in supramolecular metal complexes on a 2d superconductor. https://arxiv.org/abs/2309.02537v1 (2023).
Cai, P. et al. Visualizing the evolution from the Mott insulator to a charge-ordered insulator in lightly doped cuprates. Nat. Phys. 12 , 1047–1051 (2016).
Battisti, I. et al. Universality of pseudogap and emergent order in lightly doped Mott insulators. Nat. Phys. 13 , 21–25 (2017).
Zhong, Y. et al. Direct visualization of ambipolar Mott transition in cuprate CuO 2 planes. Phys. Rev. Lett. 125 , 077002 (2020).
Riss, A. et al. Imaging and tuning molecular levels at the surface of a gated graphene device. ACS Nano 8 , 5395–5401 (2014).
Kresse, G. & Furthmüller, J. Efficiency of ab-initio total energy calculations for metals and semiconductors using a plane-wave basis set. Comput. Mater. Sci. 6 , 15–50 (1996).
Perdew, J. P., Burke, K. & Ernzerhof, M. Generalized gradient approximation made simple. Phys. Rev. Lett. 77 , 3865–3868 (1996).
Blöchl, P. E. Projector augmented-wave method. Phys. Rev. B 50 , 17953–17979 (1994).
Kresse, G. & Joubert, D. From ultrasoft pseudopotentials to the projector augmented-wave method. Phys. Rev. B 59 , 1758–1775 (1999).
Grimme, S., Antony, J., Ehrlich, S. & Krieg, H. A consistent and accurate ab initio parametrization of density functional dispersion correction (DFT-D) for the 94 elements H-Pu. J. Chem. Phys. 132 , 154104 (2010).
Rumble, J. R. CRC handbook of chemistry and physics, 100 edn (CRC Press/Taylor & Francis, Boca Raton, FL, 2019).
Schwarz, M. et al. Corrugation in the weakly interacting hexagonal-BN/Cu(111) system: structure determination by combining noncontact atomic force microscopy and X-ray standing waves. ACS Nano 11 , 9151–9161 (2017).
Georges, A. & Kotliar, G. Hubbard model in infinite dimensions. Phys. Rev. B 45 , 6479–6483 (1992).
Georges, A. Strongly correlated electron materials: dynamical mean-field theory and electronic structure. AIP Conf. Proc. 715 , 3–74 (2004).
Parcollet, O. et al. TRIQS: a toolbox for research on interacting quantum systems. Comput. Phys. Commun. 196 , 398–415 (2015).
Seth, P., Krivenko, I., Ferrero, M. & Parcollet, O. TRIQS/CTHYB: a continuous-time quantum Monte Carlo hybridisation expansion solver for quantum impurity problems. Comput. Phys. Commun. 200 , 274–284 (2016).
Gull, E. et al. Continuous-time Monte Carlo methods for quantum impurity models. Rev. Mod. Phys. 83 , 349–404 (2011).
Kraberger, G. J., Triebl, R., Zingl, M. & Aichhorn, M. Maximum entropy formalism for the analytic continuation of matrix-valued Green’s functions. Phys. Rev. B 96 , 155128 (2017).
Bergeron, D. & Tremblay, A.-M. S. Algorithms for optimized maximum entropy and diagnostic tools for analytic continuation. Phys. Rev. E 94 , 023303 (2016).
Article ADS MathSciNet PubMed Google Scholar
Fazekas, P. Lecture notes on electron correlation and magnetism, https://www.worldscientific.com/doi/abs/10.1142/2945 (WORLD SCIENTIFIC, 1999).
Download references
Acknowledgements
A.S. acknowledges funding support from the ARC Future Fellowship scheme (FT150100426). B.J.P. acknowledges funding support from the ARC Discovery Project scheme (DP180101483). H.L.N. acknowledges funding support from the MEXT Quantum Leap Flagship Programme (JPMXS0118069605). B.L., J.H., J.C., and N.V.M. acknowledge funding support from the Australian Research Council (ARC) Centre of Excellence in Future Low-Energy Electronics Technologies (CE170100039). B.L., B.F., and J.C. are supported through Australian Government Research Training Programme (RTP) Scholarships. B.F. and N.V.M. gratefully acknowledge the computational support from the National Computing Infrastructure and Pawsey Supercomputing Facility. The authors also thank Prof. Michael S. Fuhrer, Prof. Jaime Merino Troncoso, and Dr. Daniel Moreno Cerrada for their valuable discussions.
Author information
These authors contributed equally: Benjamin Lowe, Bernard Field.
Authors and Affiliations
School of Physics and Astronomy, Monash University, Clayton, VIC, Australia
Benjamin Lowe, Bernard Field, Jack Hellerstedt, Julian Ceddia & Agustin Schiffrin
ARC Centre of Excellence in Future Low-Energy Electronics Technologies, Monash University, Clayton, VIC, Australia
Benjamin Lowe, Bernard Field, Jack Hellerstedt, Julian Ceddia, Nikhil V. Medhekar & Agustin Schiffrin
Quantum Information Science and Technology Unit, Okinawa Institute of Science and Technology Graduate University, Onna-son, Okinawa, Japan
Henry L. Nourse
School of Mathematics and Physics, The University of Queensland, Brisbane, QLD, Australia
Ben J. Powell
Department of Materials Science and Engineering, Monash University, Clayton, VIC, Australia
Nikhil V. Medhekar
You can also search for this author in PubMed Google Scholar
Contributions
B.L., J.H., and A.S. conceived and designed the experiments. B.L., J.H., and J.C. performed the experiments. B.F. and H.L.N. performed the theoretical calculations with guidance from B.J.P. and N.V.M. All authors contributed to writing the manuscript.
Corresponding authors
Correspondence to Ben J. Powell , Nikhil V. Medhekar or Agustin Schiffrin .
Ethics declarations
Competing interests.
The authors declare no competing interests.
Peer review
Peer review information.
Nature Communications thanks Ignacio Piquero-Zulaica, Amadeo L. Vázquez de Parga, and the other, anonymous, reviewer for their contribution to the peer review of this work. A peer review file is available.
Additional information
Publisher’s note Springer Nature remains neutral with regard to jurisdictional claims in published maps and institutional affiliations.
Supplementary information
Supplementary information, peer review file, rights and permissions.
Open Access This article is licensed under a Creative Commons Attribution 4.0 International License, which permits use, sharing, adaptation, distribution and reproduction in any medium or format, as long as you give appropriate credit to the original author(s) and the source, provide a link to the Creative Commons licence, and indicate if changes were made. The images or other third party material in this article are included in the article’s Creative Commons licence, unless indicated otherwise in a credit line to the material. If material is not included in the article’s Creative Commons licence and your intended use is not permitted by statutory regulation or exceeds the permitted use, you will need to obtain permission directly from the copyright holder. To view a copy of this licence, visit http://creativecommons.org/licenses/by/4.0/ .
Reprints and permissions
About this article
Cite this article.
Lowe, B., Field, B., Hellerstedt, J. et al. Local gate control of Mott metal-insulator transition in a 2D metal-organic framework. Nat Commun 15 , 3559 (2024). https://doi.org/10.1038/s41467-024-47766-8
Download citation
Received : 13 July 2023
Accepted : 10 April 2024
Published : 26 April 2024
DOI : https://doi.org/10.1038/s41467-024-47766-8
Share this article
Anyone you share the following link with will be able to read this content:
Sorry, a shareable link is not currently available for this article.
Provided by the Springer Nature SharedIt content-sharing initiative
By submitting a comment you agree to abide by our Terms and Community Guidelines . If you find something abusive or that does not comply with our terms or guidelines please flag it as inappropriate.
Quick links
- Explore articles by subject
- Guide to authors
- Editorial policies
Sign up for the Nature Briefing newsletter — what matters in science, free to your inbox daily.

Metal-Organic Framework-Enabled Sustainable Agrotechnologies: An Overview of Fundamentals and Agricultural Applications
Affiliations.
- 1 State Key Laboratory for Crop Stress Resistance and High-Efficiency Production, Northwest A&F University, Yangling, Shaanxi 712100, People's Republic of China.
- 2 College of Food Science and Engineering, Northwest A&F University, Yangling, Shaanxi 712100, People's Republic of China.
- 3 Department of Plant and Soil Sciences, University of Kentucky, Lexington, Kentucky 40546, United States.
- 4 Kentucky Water Resources Research Institute, University of Kentucky, Lexington, Kentucky 40506, United States.
- PMID: 38600745
- DOI: 10.1021/acs.jafc.4c00764
With aggravated abiotic and biotic stresses from increasing climate change, metal-organic frameworks (MOFs) have emerged as versatile toolboxes for developing environmentally friendly agrotechnologies aligned with agricultural practices and safety. Herein, we have explored MOF-based agrotechnologies, focusing on their intrinsic properties, such as structural and catalytic characteristics. Briefly, MOFs possess a sponge-like porous structure that can be easily stimulated by the external environment, facilitating the controlled release of agrochemicals, thus enabling precise delivery of agrochemicals. Additionally, MOFs offer the ability to remove or degrade certain pollutants by capturing them within their pores, facilitating the development of MOF-based remediation technologies for agricultural environments. Furthermore, the metal-organic hybrid nature of MOFs grants them abundant catalytic activities, encompassing photocatalysis, enzyme-mimicking catalysis, and electrocatalysis, allowing for the integration of MOFs into degradation and sensing agrotechnologies. Finally, the future challenges that MOFs face in agrotechnologies were proposed to promote the development of sustainable agriculture practices.
Keywords: absorbents; agrotechnologies; catalysts; metal−organic frameworks; slow release.
Publication types

Maintenance work is planned for Wednesday 1st May 2024 from 9:00am to 11:00am (BST).
During this time, the performance of our website may be affected - searches may run slowly and some pages may be temporarily unavailable. If this happens, please try refreshing your web browser or try waiting two to three minutes before trying again.
We apologise for any inconvenience this might cause and thank you for your patience.
Inorganic Chemistry Frontiers
Surface functionalization of metal-organic framework nanoparticle for overcoming biological barrier in cancer therapy.
Nanomedicine, which merges the realms of nanotechnology and medicine, presents transformative strategies for advancing healthcare through nanoscale manipulation of materials. Among these, metal–organic frameworks (MOFs), with a unique hybrid structure of metal ions interconnected by organic ligands, have emerged as a novel class of inorganic nanoparticles with significant potential in drug delivery systems (DDS). This study explores the innovative applications of MOFs in DDS, emphasizing their distinctive porous architectures that facilitate the adsorption and controlled release of therapeutic agents. The versatility of MOFs is further enhanced by surface functionalization, which not only augments their dispersibility and targeting efficiency but also addresses the biological barriers that traditionally impede nanoparticle-based drug delivery. These barriers include the adsorption of bioproteins, which can mask the nanoparticles and prompt immune recognition, and the complex interplay within the tumor microenvironment (TME), which affects nanoparticle penetration and efficacy. Additionally, the cell membrane prevents nanoparticles from being internalized into the cell, and drugs released within the cell are removed by efflux pumps, impeding their therapeutic effect. Through a comprehensive analysis, we highlighted the strategies employed to overcome these obstacles, leveraging the multifunctional capabilities of MOFs to enhance their therapeutic payload delivery and targeting precision.
- This article is part of the themed collections: 2024 Inorganic Chemistry Frontiers HOT articles and 2024 Inorganic Chemistry Frontiers Review-type Articles
Article information

Download Citation
Permissions.

J. Y. Oh, Y. Sim, G. Yang, M. Park, K. Kim and J. Ryu, Inorg. Chem. Front. , 2024, Accepted Manuscript , DOI: 10.1039/D4QI00523F
This article is licensed under a Creative Commons Attribution 3.0 Unported Licence . You can use material from this article in other publications without requesting further permissions from the RSC, provided that the correct acknowledgement is given.
Read more about how to correctly acknowledge RSC content .
Social activity
Search articles by author.
This article has not yet been cited.
Advertisements
Help | Advanced Search
Condensed Matter > Materials Science
Title: harnessing large language model to collect and analyze metal-organic framework property dataset.
Abstract: This research was focused on the efficient collection of experimental Metal-Organic Framework (MOF) data from scientific literature to address the challenges of accessing hard-to-find data and improving the quality of information available for machine learning studies in materials science. Utilizing a chain of advanced Large Language Models (LLMs), we developed a systematic approach to extract and organize MOF data into a structured format. Our methodology successfully compiled information from more than 40,000 research articles, creating a comprehensive and ready-to-use dataset. The findings highlight the significant advantage of incorporating experimental data over relying solely on simulated data for enhancing the accuracy of machine learning predictions in the field of MOF research.
Submission history
Access paper:.
- Other Formats

References & Citations
- Google Scholar
- Semantic Scholar
BibTeX formatted citation

Bibliographic and Citation Tools
Code, data and media associated with this article, recommenders and search tools.
- Institution
arXivLabs: experimental projects with community collaborators
arXivLabs is a framework that allows collaborators to develop and share new arXiv features directly on our website.
Both individuals and organizations that work with arXivLabs have embraced and accepted our values of openness, community, excellence, and user data privacy. arXiv is committed to these values and only works with partners that adhere to them.
Have an idea for a project that will add value for arXiv's community? Learn more about arXivLabs .
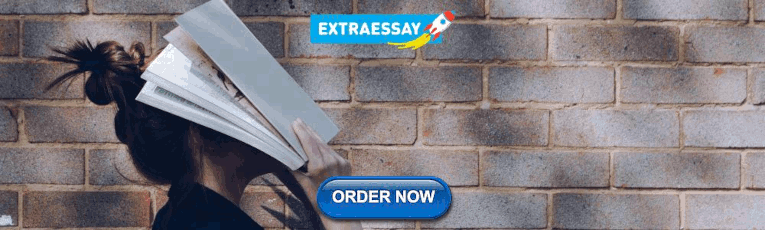
IMAGES
VIDEO
COMMENTS
Metal ions or clusters that have been bonded with organic linkers to create one- or more-dimensional structures are referred to as metal-organic frameworks (MOFs). Reticular synthesis also forms MOFs with properly designated components that can result in crystals with high porosities and great chemical and thermal stability. Due to the wider surface area, huge pore size, crystalline nature ...
Metal organic frameworks (MOFs) are considered as a group of compounds, either metal ions or clusters, harmonized with organic ligands to form one or some dimensional structures. ... However, literature review showed that some regular structural motifs in MOF preparation existed which is an attempt to predict their architectures [13], [14], ...
Metal-organic frameworks (MOFs) possess excellent advantages, such as high porosity, large specific surface area, and an adjustable structure, showing good potential for applications in gas adsorption and separation, catalysis, conductivity, sensing, magnetism, etc. However, they still suffer from significant limitations in terms of the scale-up synthesis and shaping, hindering the ...
Metal-organic frameworks (MOFs) are a class of porous materials with unprecedented chemical and structural tunability. Their synthetic versatility, long-range order, and rich host-guest ...
Metal-organic frameworks (MOFs), also known as porous coordination polymers (PCPs), are constructed by organic linkers and metal ions or clusters and have emerged as a new type of crystalline materials with large surface area (typically ranging from 1000 to 10,000 m 2 /g), high porosity, tunable structures, and flexible tailorability, compared with traditional porous materials such as ...
Abstract. Metal organic frameworks (MOFs) are considered as a group of compounds, either metal ions or clusters, harmonized with organic ligands to form one or some dimensional structures. In addition to resilient bonds between inorganic and organic units, reticular synthesis creates MOFs, accurate selection of constituents of which can produce ...
Background. Metal-organic frameworks (MOFs) are made by linking inorganic and organic units by strong bonds (reticular synthesis). The flexibility with which the constituents' geometry, size, and functionality can be varied has led to more than 20,000 different MOFs being reported and studied within the past decade.
1. Introduction. Metal-organic frameworks (MOFs) are produced by the formation of chemical bonds between organic ligands as linkers and metal ions as nodes, leading to generation of periodic network crystalline structures with high porosities and large surface areas, which promote their potential applications in various fields of science and technology. 1 In recent years, absorbent materials ...
Metal-organic frameworks (MOFs) are an emerging class of porous materials created by the assembly of inorganic connectors and organic linkers. They have potential applications in fields such as gas storage as well as separation, sensing, catalysis, and drug delivery due to its properties such as flexibility, porosity, high surface area and functionality. Among the various synthetic ...
Fan et al. [30] performed microwave-assisted syn-thesis of zirconium-based metal-organic frameworks. They compared the yield and porosity by changing quantity of modulator, reaction time and temperature. The reaction was completed in 2-2.5 h in microwave which took 24 h for completion in solvothermal method.
Metal-organic frameworks (MOFs) are a type of organic-inorganic hybrid material with a distinctive 3D network crystal structure. Lately, MOFs have made striking progress in the fields of adsorption, catalytic degradation, and biomedicine on account of their large specific surface and well-developed pore structure.
As a library, NLM provides access to scientific literature. Inclusion in an NLM database does not imply endorsement of, or agreement with, the contents by NLM or the National Institutes of Health. ... A Comprehensive Review of Metal-Organic Framework: Synthesis, Characterization, and Investigation of Their Application in Electrochemical ...
Metal organic framework is a class of hybrid network of supramolecular solid materia ls comprised of a large. number of inorganic and organic linkers all bounded to metal ions in a well organised ...
In this Review we survey the molecular sieving behaviour of metal-organic framework (MOF) and covalent organic framework (COF) membranes, which is different from that of classical zeolite membranes.
Metal-organic frameworks (MOFs), which are constructed by metal ions or clusters with organic ligands, have shown great potential in gas storage and separation, luminescence, catalysis, drug delivery, sensing, and so on. More than 20,000 MOFs have been reported by adjusting the composition and reaction conditions, and most of them were synthesized by hydrothermal or solvothermal methods. The ...
* Corresponding authors a College of Chemistry and Chemical Engineering, Qingdao University, Shandong 266071, China E-mail: [email protected], [email protected] b School of Materials Science and Engineering, Smart Sensing Interdisciplinary Science Centre, TKL of Metal and Molecule-Based Material Chemistry, Nankai University, Tianjin 300350, China
Metal-organic frameworks (MOFs) are intrinsically porous extended solids formed by coordination bonding between organic ligands and metal ions or clusters. High electrical conductivity is rare in MOFs, yet it allows for diverse applications in electrocatalysis, charge storage, and chemiresistive sensing, among others. In this Review, we discuss the efforts undertaken so far to achieve ...
Abstract. The third generation of metal-organic frameworks responds to the advent of a guest molecule and on the application of external stimuli such as temperature, light, mechanical stress, and pressure. They are used in separation, sensing, drug delivery, gas storage, catalysis, etc due to their ability to make adjustments in their pore size.
This literature review demonstrates that metal-organic frameworks can be promising adsorbents for indoor air cleaning. The green synthesis methods, stability, adsorption performance under low concentration and diverse VOCs conditions, and application methods for metal-organic frameworks should be further researched before their large-scale ...
Introduction. Metal-Organic Frameworks (MOFs) or porous coordination networks are a class of advanced materials designed by employing various metal ions and organic linkers. MOFs have emerged as an extensive class of crystalline materials with high porosity in nature. Unlike other porous materials like zeolites, activated carbon, and metal ...
The application of MOFs as advanced materials and systems for cancer therapy is the main scope beyond this perspective. Some challenging aspects and promising features in MOF-based cancer diagnosis and cancer therapy have also been discussed. Keywords: metal-organic frameworks (MOFs), cancer therapy, biotechnology, nanomedicine. Go to: 1.
The electronic correlation-driven Mott metal-insulator transition has been predicted in a 2D metal-organic framework with a kagome structure. Here the authors synthesize such a system in ...
With aggravated abiotic and biotic stresses from increasing climate change, metal-organic frameworks (MOFs) have emerged as versatile toolboxes for developing environmentally friendly agrotechnologies aligned with agricultural practices and safety. Herein, we have explored MOF-based agrotechnologies, focusing on their intrinsic properties, such ...
Metal-organic frameworks (MOFs), as a class of crystalline porous coordinated materials, have become significant candidates in various applications through their outstanding characteristics such as high surface area, large pore size, tunable porosity, and high thermal stability. Therefore, MOFs are capable of being used as heterogeneous catalysts directly, or as carriers for homogenous ...
Nanomedicine, which merges the realms of nanotechnology and medicine, presents transformative strategies for advancing healthcare through nanoscale manipulation of materials. Among these, metal-organic frameworks (MOFs), with a unique hybrid structure of metal ions interconnected by organic ligands, have eme 2024 Inorganic Chemistry Frontiers HOT articles 2024 Inorganic Chemistry Frontiers ...
View PDF Abstract: This research was focused on the efficient collection of experimental Metal-Organic Framework (MOF) data from scientific literature to address the challenges of accessing hard-to-find data and improving the quality of information available for machine learning studies in materials science. Utilizing a chain of advanced Large Language Models (LLMs), we developed a systematic ...
The combination of single-ion magnets (SIMs) and metal-organic frameworks (MOFs) is expected to produce new quantum materials. The principal issue to be solved in this regard is the development of ...