A protein assembly-disassembly pathway in vitro that may correspond to sequential steps of synaptic vesicle docking, activation, and fusion
Affiliation.
- 1 Program in Cellular Biochemistry and Biophysics, Memorial Sloan-Kettering Cancer Center, New York, New York 10021.
- PMID: 8221884
- DOI: 10.1016/0092-8674(93)90376-2
The SNARE hypothesis holds that a transport vesicle chooses its target for fusion when a soluble NSF attachment protein (SNAP) receptor on the vesicle (v-SNARE) pairs with its cognate t-SNARE at the target membrane. Three synaptosomal membrane proteins have previously been identified: syntaxin, SNAP-25 (t-SNAREs), and vesicle-associated membrane protein (VAMP) (v-SNARE); all assemble with SNAPs and NSF into 20S fusion particles. We now report that in the absence of SNAP and NSF, these three SNAREs form a stable complex that can also bind synaptotagmin. Synaptotagmin is displaced by alpha-SNAP, suggesting that these two proteins share binding sites on the SNARE complex and implying that synaptotagmin operates as a "clamp" to prevent fusion from proceeding in the absence of a signal. The alpha-SNAP-SNARE complex can bind NSF, and NSF-dependent hydrolysis of ATP dissociates the complex, separating syntaxin, SNAP-25, and VAMP. ATP hydrolysis by NSF may provide motion to initiate bilayer fusion.
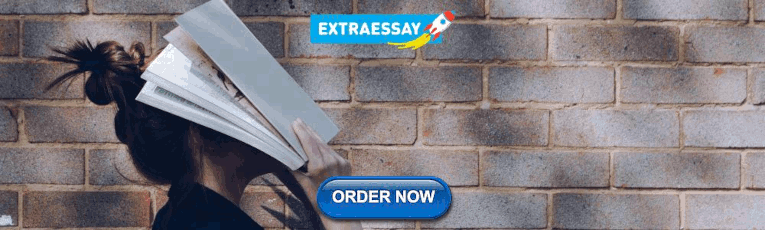
Publication types
- Research Support, Non-U.S. Gov't
- Research Support, U.S. Gov't, P.H.S.
- Adenosine Triphosphatases / metabolism
- Antigens, Surface / isolation & purification
- Brain / metabolism
- Calcium-Binding Proteins*
- Carrier Proteins / metabolism
- Fluorescent Antibody Technique
- Membrane Fusion*
- Membrane Glycoproteins / metabolism
- Membrane Proteins*
- Models, Biological
- N-Ethylmaleimide-Sensitive Proteins
- Nerve Tissue Proteins / isolation & purification
- Nerve Tissue Proteins / metabolism*
- Synaptic Vesicles / metabolism*
- Synaptosomal-Associated Protein 25
- Synaptotagmins
- Vesicular Transport Proteins*
- Antigens, Surface
- Calcium-Binding Proteins
- Carrier Proteins
- Membrane Glycoproteins
- Membrane Proteins
- Nerve Tissue Proteins
- Vesicular Transport Proteins
- Adenosine Triphosphatases
- Protein family review
- Published: 24 October 2001
The syntaxins
- Felicia Yu Hsuan Teng 1 ,
- Ya Wang 1 &
- Bor Luen Tang 1 , 2
Genome Biology volume 2 , Article number: reviews3012.1 ( 2001 ) Cite this article
16k Accesses
104 Citations
3 Altmetric
Metrics details
The SNARE hypothesis predicts that a family of SNAP receptors are localized to and function in diverse intracellular membrane compartments where membrane fusion processes take place. Syntaxins, the prototype family of SNARE proteins, have a carboxy-terminal tail-anchor and multiple coiled-coil domains. There are 15 members of the syntaxin family in the human genome and 7 syntaxin-like genes in the yeast Saccharomyces cerevisiae. In conjunction with other SNAREs and with the cytoplasmic NSF and SNAP proteins, syntaxins mediate vesicle fusion in diverse vesicular transport processes along the exocytic and the endocytic pathway. They are crucial components that both drive and provide specificity to the myriad vesicular fusion processes that characterize the eukaryotic cell.
Traffic between intracellular membrane compartments is largely mediated by vesicular transport. High degrees of specificity and complexity are exerted in the regulation of vesicle budding, docking and fusion. In a breakthrough, Rothman and colleagues showed in a cell-free assay that the docking and fusion of transport vesicles require the concerted action of two cytosolic proteins: N -ethylmaleimide-sensitive factor (NSF), an ATPase whose activity regulates the formation and dissociation of the complexes needed for membrane fusion, and an additional factor needed to attach NSF to Golgi membranes, the soluble NSF-attachment protein, SNAP (reviewed in [ 1 ]).
Factors that determine the specificity of the docking and fusion of vesicles to the correct target membranes were subsequently identified from their incorporation, again in a cell-free assay, into a 20S assembly complex with recombinant NSF and α-SNAP [ 2 ]. These SNAP receptors, or SNAREs, turned out to be previously cloned components of the synaptic membrane with unknown function. Remarkably, these SNAREs share structural homology with several yeast genes whose products are associated with vesicular transport. These findings pointed towards a conserved mechanism in the diverse transport processes amongst eukaryotic cells. To account for the specificity of each step, the SNARE hypothesis [ 3 ] postulated the existence of a family of molecules, with each molecule functioning in one of the various membrane-fusion processes in the cell. One of the molecules identified as a SNARE from bovine brain was a syntaxin.
Gene organization and evolutionary history
Syntaxin was first described as two 35 kDa proteins (now known as syntaxin 1A and 1B), 84% identical to each other in amino-acid sequence, that interact with the synaptic-vesicle protein synaptotagmin [ 4 ]. It soon became clear that there are also non-neuronal homologs of syntaxin. The first syntaxin localized to the early secretory pathway, syntaxin 5, was cloned along with the cell-surface syntaxins 2, 3 and 4 [ 5 ]. More recently, syntaxins localized to the endosomes have also been identified [ 6 ]. A summary of the genetic, cellular and functional information of known mammalian and yeast syntaxins and their phylogenetic relationships is shown in Tables 1 and 2 and Figure 1 .
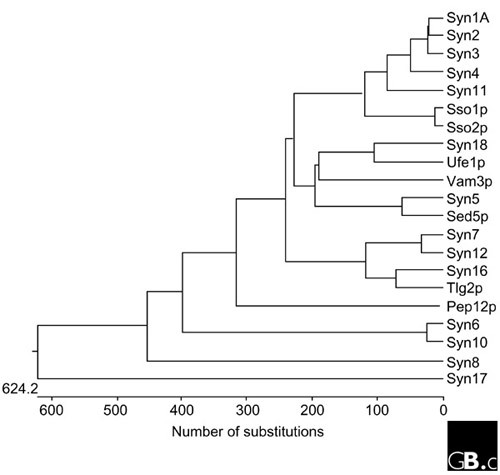
A nearest-neighbor dendrogram of the 7 S. cerevisiae and 15 mammalian syntaxins, generated with the DNASTAR program. See Table 2 for yeast gene names; syn, syntaxin. There are also several syntaxin-like genes in the Drosophila and C. elegans genome; see Bock et al. [6] for a more extensive phylogenetic analysis that includes these sequences.
The syntaxin family consists of 15 genes in mammals and 7 in yeast, and there are syntaxin-like sequences in all eukaryotes examined to date. The mammalian syntaxin genes are on different chromosomes (summarized in Table 1 ). Syntaxins 3 and 5 are both found on chromosome 11 but at different loci. Additional diversity within the syntaxin family is generated by alternative splicing; alternatively spliced isoforms have been identified for syntaxins 1A, 2, 3, 5 and 16. The domains that can be removed or included by alternative splicing commonly include the membrane-proximal domain of syntaxin, the region required for SNARE complex assembly and/or the carboxy-terminal hydrophobic membrane anchor. The splice isoforms are differentially expressed during development and in different tissues in adult life, and may thus have substantially different functional roles in the regulation of membrane traffic.
Characteristic structural features
All mammalian syntaxins, with the exception of syntaxin 11, are transmembrane proteins anchored by their carboxy-terminal tails with a type II orientation (that is, with the amino terminus and the bulk of the polypeptide facing the cytoplasm). The domain structure of syntaxin 1A, the first to be identified, is shown schematically in Figure 2b . Other than the transmembrane domain, there are several hydrophobic regions (Figure 2a ) with the potential to form coiled-coil α-helical structures. The approximately 60-residue-long membrane-proximal coiled-coil domain is the SNARE domain, which is characteristic of and conserved in all syntaxins [ 7 ].
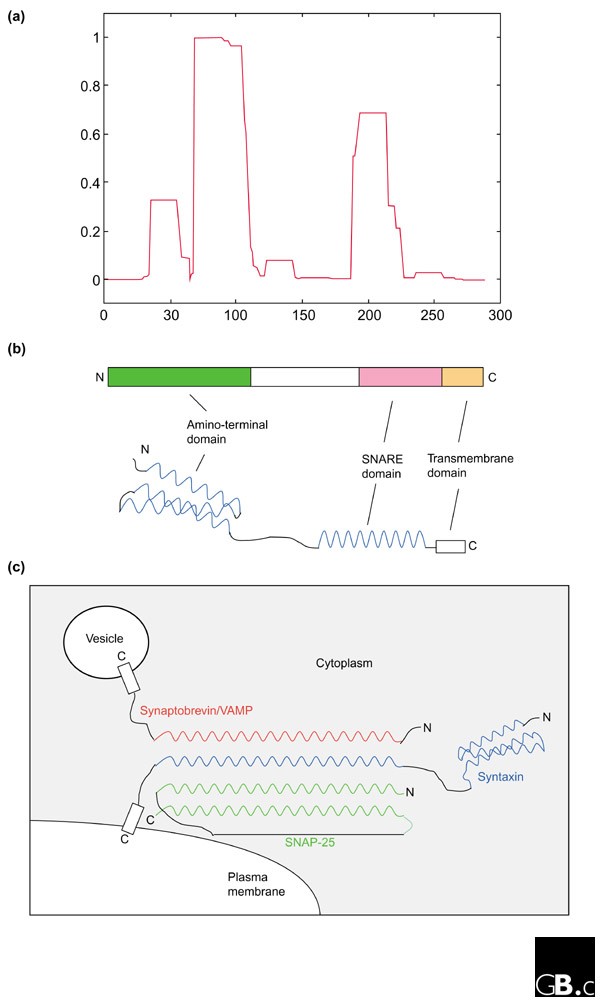
The structure of syntaxin and a syntaxin-containing SNARE complex. (a) A graphical output of the analysis with the COILS program [16] of syntaxin 1A, for potential coiled-coil-forming regions. A window size of 21 residues was used. (b) Schematic representation of the structure of syntaxin 1A, illustrating both the linear domain arrangement (upper diagram) and the coiled-coil domains (blue) with spatial relevance to one another (lower diagram). N, amino terminus; C, carboxyl terminus. (c) Schematic representation of the four-helical bundle structure of the core fusion complex formed by syntaxin 1A (blue), synaptobrevin/VAMP (red) and SNAP-25 (green) at the presynaptic plasma membrane.
The SNARE domain of a syntaxin mediates its interactions with the SNARE domains of other target-membrane (t) SNARE proteins from the syntaxin or SNAP-25 families, to form t-SNARE complexes at target membranes. The t-SNARE complexes, in turn, interact with SNARE domains of the vesicle (v) SNAREs (vesicle-associated membrane proteins, VAMPs) found on specific vesicle membranes, to form the core fusion complex. Perhaps the best-studied membrane-fusion complex is that mediating synaptic-vesicle fusion [ 8 , 9 , 10 ]. An extremely stable ternary complex, a 12 nm long twisted bundle of four helices aligned in parallel, is formed by syntaxin 1A, SNAP-25 and VAMP-2, which contribute one, two and one α helices, respectively (Figure 2c ) [ 11 ]. Most other heterotypic core fusion complexes are likely to have a similar parallel four-helical bundle structure, but in some cases the two α-helical SNARE domains provided by SNAP-25 may be replaced by the SNARE domains of two members of the syntaxin family.
The amino terminus of some syntaxins, such as syntaxin 1, contains another characteristic domain, which is thought to vary between syntaxin isoforms depending on the specific vesicle-traffic steps involved. The amino-terminal domain of syntaxin 1 (Figure 2b ) is a bundle of three α helices with a left-handed twist [ 12 ]. This conserved autonomously folding amino-terminal structure may serve as an auto-inhibitory regulatory domain. By folding back onto the membrane-proximal SNARE domain, the molecule adopts a 'closed' configuration that prevents the formation of the core fusion complex. The chaperone protein n-Sec1/Munc18 binds to this closed conformation of syntaxin. Dissociation or conformational changes in n-Sec1/Munc-18 induced by the Rab small GTPases may open up the structure to facilitate SNARE-complex formation.
SNARE complex assembly and disassembly
SNAREs, together with SNAP and NSF, form a 20S complex intermediate that is essential for the docking and fusion of vesicles with a target membrane. As mentioned, in the case of synaptic-vesicle exocytosis, syntaxin 1 and its cognate SNARE partners form a ternary complex consisting of a coiled-coil bundle of four α helices, before NSF/α-SNAP joins to form the 20S complex. These four helices are parallel with the transmembrane domains of VAMP and syntaxin, at the same end of the bundle. The formation of the 20S complex thus creates a bridge between the vesicle and the target membrane. Evidence suggests that, in the initial stage of vesicle docking, the SNARE complex assumes a partial and reversible assembly known as the ' trans -conformation'. In this case, the syntaxin coil is likely to be less tightly associated than the v-SNARE and SNAP25 coils, and its full association is postulated to be held back by a calcium sensor, until the arrival of Ca 2+ signal. The Ca 2+ trigger results in the full association of the syntaxin coil, converting the trans -complex into a tight ' cis -complex'. In a 'zipper' model of SNARE-mediated fusion, it is postulated that the trans -complex might zipper up from the amino terminus towards the carboxy-terminal transmembrane end, bringing the two membranes together and thereby causing the final membrane fusion.
The cis -complex formed after vesicle docking and membrane fusion is then dissociated by NSF and its co-factor α-SNAP. The hexameric NSF has two ATP-binding sites (D1 and D2) per subunit. The binding of α-SNAP to NSF stimulates nucleotide hydrolysis at the D1 sites. NSF undergoes a conformational change upon ATP hydrolysis, which provides a mechanical force for disassembling the SNARE complex. The disassembly of the complex by NSF frees the SNAREs for recycling and the formation of a new trans -complex for the next round of vesicle docking and fusion.
Localization and function
A summary of the cellular localization of mammalian and yeast syntaxins can be found in Tables 1 and 2 and Figure 3 . Cellular localizations for all mammalian syntaxins have been confirmed using antibodies against endogenous proteins. It is worth noting that, although transgenic syntaxins epitope-tagged at the amino terminus generally retain the localization of the endogenous protein, overflowing of the proteins to neighboring compartments as a result of overexpression can complicate interpretation of localization experiments. On the other hand, morphological changes to particular cellular compartments - due to transport defects or otherwise - as a result of overexpression of a particular syntaxin or its mutant can also be informative with regard to its localization and site of function.
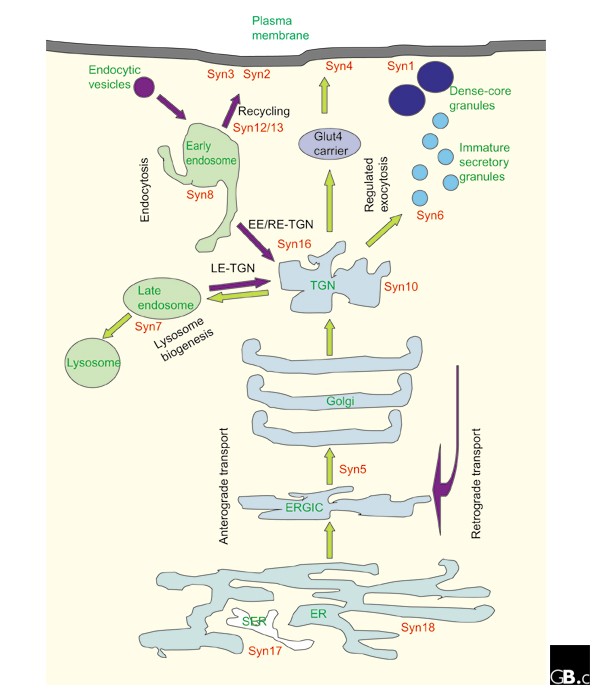
Subcellular localization of syntaxins (red) in a mammalian cell relative to the various membrane-bound compartments, anterograde and endocytotic/retrograde flow of traffic (green and purple arrows, respectively) and known membrane-transport steps (black). EE, early endosome; ERGIC, ER-Golgi intermediate compartment; Glut4, a glucose transporter molecule; LE, late endosome; RE, recycling endosome, SER, smooth ER; syn, syntaxin; TGN, trans -Golgi network.
The transmembrane tail anchor found in all syntaxins except syntaxin 11 is essential for their membrane localization (exogenously expressed cytoplasmic domains appear to be cytosolic), but in most cases it is not sufficient for specific targeting to particular membranes. Compartmental targeting signals reside in the cytoplasmic domains, and these are not well defined. An examination of the amino-acid sequences of syntaxins that are found in the trans -Golgi network (TGN) and post-Golgi compartments has revealed putative targeting signals with dileucine motifs, but these have not, in most cases, been shown to be functional by mutational analysis.
The SNARE hypothesis predicts that, as a t-SNARE, the cellular function of a particular syntaxin would logically be determined and restricted by its localization. Thus, it is not surprising to find that syntaxin 18, which is localized to the endoplasmic reticulum (ER), and the cis -Golgi-localized syntaxin 5 have roles in ER-to-Golgi transport. In fact, the lack of a syntaxin within the Golgi stack itself has been suggested as indirect evidence for the maturation model of intra-Golgi transport (in which Golgi compartments move from ER to TGN as they mature, as opposed to the vesicular transport model, in which vesicles shunt between 'static' compartments). On the other hand, one explanation for the apparent multiple functions of the predominantly TGN-localized syntaxin 6 is that it is perhaps not a true syntaxin, even though it has been categorized as one, as its SNARE domain resembles that of the SNAP23/25/29 family members more than the syntaxins [ 6 ]. The exact roles of the endosomal syntaxins (syntaxin 7, 8, 11 and 12/13) are not particularly well defined. One reason for this is the lack of biochemical assays to precisely dissect the complicated steps in endosomal transport. The surface syntaxins have been implicated in various processes involving the delivery of TGN cargo to the cell surface. Syntaxin 4, for example, is essential for translocation of the glucose transporter molecule Glut4 to the plasma membrane of insulin-responsive cells.
Syntaxins have been shown to interact with a range of other proteins as well as their SNARE partners. These can be broadly classified as either components of the vesicular transport machinery or proteins with no predicted function in vesicular transport. The former include vesicle coat proteins, Rab GTPases and tethering factors. It would not be surprising or exceptionally interesting that syntaxins may interact with coat proteins, which are, after all, cargo proteins in their own right. There is evidence, however, that some v-SNAREs are initiators of coat-protein assembly and vesicle budding. This makes sense as it would ensure that vesicles are 'functional', equipped with downstream docking and fusion components. Rab proteins and their effectors are regulators of the vesicle docking and fusion processes. Multiprotein complexes such as the homotypic fusion and vacuole protein sorting (HOPS) complex interact functionally with Rabs and SNAREs to regulate docking [ 13 ]. In perhaps most instances, vesicle docking is preceded by a process known as tethering, whereby molecules known as tethering factors bring vesicles close to the target membrane, to enhance productive docking and fusion. The direct interaction between a syntaxin, syntaxin 13, and the tethering factor early endosome antigen 1 (EEA1) has been elegantly demonstrated in the case of Rab5-regulated early endosomal homotypic fusion (fusion of similar vesicles) [ 14 ].
Syntaxins may also interact with other proteins. Syntaxin 1A and syntaxin 3 have been known to interact with sodium channels in epithelial cells, thereby regulating the intrinsic properties and cell-surface expression of the channels. An antibody against syntaxin 1A immunoprecipitates solubilized N-type calcium channels, suggesting a role in docking synaptic vesicles near calcium channels in presynaptic active zones. The interaction between syntaxin 1A and voltage-sensitive calcium channels in an 'excitosome' complex at the presynaptic plasma membrane may enable a rapid secretory response to a membrane-depolarizing signal.
With the solution of the structures of SNARE complexes and advanced biochemical and biophysical analysis, we now have a fair idea of how syntaxins interact with their SNARE partners and how these interactions are regulated. With the completion of the sequencing of the human genome, all human syntaxins have now been identified. Many of the cellular and physiological functions of syntaxins remain to be learned, however.
Firstly, the exact components of the SNARE complexes, or for that matter the exact involvement of any particular syntaxin, remains unclear in several important membrane transport steps. It is not yet known, for example, whether syntaxin 18 has a function in homotypic fusion in the ER, like the yeast homolog Ufe1p. The mammalian syntaxin 5 appears to be present both on vesicles en route to the Golgi and on the target membrane itself, and can be found in distinct SNARE complexes. It is not known if the SNARE complexes that include syntaxins 5 and 18 function in sequence or in parallel in ER-Golgi transport. Likewise, syntaxin 6 appears to serve multiple functions in transport processes in and out of the TGN that are not yet clearly defined, as well as post-TGN processes. As already mentioned, much remains to be learned about the mechanistic roles of the endosomal syntaxins. Another important line of investigation is to confirm the participation of the cell-surface syntaxins (especially syntaxin 3) in specialized processes such as neurite outgrowth and myelin sheath formation.
Secondly, although it is clear that syntaxins may have physiologically important direct or indirect physical interactions with other components of the vesicular transport system that do not belong to the core machinery of membrane fusion, much of this knowledge is fragmented. The interactions of syntaxins with Rab proteins and tethering proteins have been investigated and demonstrated only in isolated cases. Extension of this type of knowledge to other syntaxins is absolutely essential for our further understanding of the regulation of syntaxin function. Also, reports of interactions between syntaxins and other molecules that do not appear to serve general roles in transport are confined to the ion channels that interact with syntaxin 1 and 3. Further efforts in looking for interactions of syntaxins with non-SNARE and non-transport components are warranted.
Finally, the functions of most of the syntaxins with respect to organism growth, physiology and development are absolutely unknown. It would be fair to speculate that syntaxins in the early secretory pathway of mammalian cells would be so vital that any ablation of their genes would result in death of cells, let alone of the organism. But the fact that all post-Golgi syntaxin-like molecules in yeast are not essential for growth points to the possibility that some of the mammalian post-Golgi syntaxins may have physiological functions that are amenable to genetic analysis by mouse 'knockout' and 'knockin' genetic approaches. If so, it may then be possible to investigate whether these syntaxins have a role in embryonic development, post-natal growth, or the organization of particular tissues or structures. Extracting mechanistic information from the knockout phenotypes may be difficult, though. There is clearly much more to learn about the physiological functions of these important proteins.
Rothman JE: Mechanisms of intracellular protein transport. Nature. 1994, 372: 55-63. 10.1038/372055a0. A review covering most of the early mechanistic findings on vesicular transport.
Article PubMed CAS Google Scholar
Sollner T, Whiteheart SW, Brunner M, Erdjument-Bromage H, Geromanos S, Tempst P, Rothman JE: SNAP receptors implicated in vesicle targeting and fusion. Nature. 1993, 362: 318-324. 10.1038/362318a0. A landmark paper describing the purification and identification of SNAP receptors, or SNAREs.
Rothman JE, Warren G: Implications of the SNARE hypothesis for intracellular membrane topology and dynamics. Curr Biol. 1994, 4: 220-233. An elaborate account of the SNARE hypothesis, in which the authors propose that multiple SNAREs at various membranes provide the specificity for vesicular transport.
Bennett MK, Calakos N, Scheller RH: Syntaxin: a synaptic protein implicated in docking of synaptic vesicles at presynaptic active zones. Science. 1992, 257: 255-259. The first cloning of a syntaxin.
Bennett MK, Garcia-Arraras JE, Elferink LA, Peterson K, Fleming AM, Hazuka CD, Scheller RH: The syntaxin family of vesicular transport receptors. Cell. 1993, 74: 863-873. Cloning of the first non-neuronal syntaxins.
Bock JB, Matern HT, Peden AA, Scheller RH: A genomic perspective on membrane compartment organization. Nature. 2001, 409: 839-841. 10.1038/35057024. A detailed and thorough analysis of transport-related proteins in the sequenced genomes. An excellent reference source for syntaxins.
Weimbs T, Low SH, Chapin SJ, Mostov KE, Bucher P, Hofmann K: A conserved domain is present in different families of vesicular fusion proteins: a new superfamily. Proc Natl Acad Sci USA. 1997, 94: 3046-3051. 10.1073/pnas.94.7.3046. A classic bioinformatics paper that provided the first idea of how syntaxins and VAMPs are related.
Article PubMed CAS PubMed Central Google Scholar
Chen YA, Scheller RH: SNARE-mediated membrane fusion. Nat Rev Mol Cell Biol. 2001, 2: 98-106. 10.1038/35052017. The most up-to-date review on the mechanisms of SNARE-mediated membrane fusion.
Jahn R, Sudhof TC: Membrane fusion and exocytosis. Annu Rev Biochem. 1999, 68: 863-911. 10.1146/annurev.biochem.68.1.863. A detailed review of the players and mechanisms involved in exocytosis.
Lin RC, Scheller RH: Mechanisms of synaptic vesicle exocytosis. Annu Rev Cell Dev Biol. 2000, 16: 19-49. 10.1146/annurev.cellbio.16.1.19. A detailed review of the players and mechanisms of synaptic-vesicle exocytosis.
Sutton RB, Fasshauer D, Jahn R, Brunger AT: Crystal structure of a SNARE complex involved in synaptic vesicle exocytosis at 2.4 Å resolution. Nature. 1998, 395: 347-353. 10.1038/26412. The first description of the crystal structure of a SNARE complex.
Lerman JC, Robblee J, Fairman R, Hughson FM: Structural analysis of the neuronal SNARE protein syntaxin-1A. Biochemistry. 2000, 39: 8470-8479. 10.1021/bi0003994. Structural analysis of the amino-terminal domain of syntaxin 1A at 1.9Å resolution.
Eitzen G, Will E, Gallwitz D, Haas A, Wickner W: Sequential action of two GTPases to promote vacuole docking and fusion. EMBO J. 2000, 19: 6713-6720. 10.1093/emboj/19.24.6713. Vacuole homotypic fusion in yeast is perhaps the system in which membrane fusion mechanisms have been most studied. This paper, one of an excellent series from William Wickner's laboratory, describes how Rab GTPases regulate the process.
McBride HM, Rybin V, Murphy C, Giner A, Teasdale R, Zerial M: Oligomeric complexes link Rab5 effectors with NSF and drive membrane fusion via interactions between EEA1 and syntaxin 13. Cell. 1999, 98: 377-386. An important paper showing a direct link between a Rab protein, a tethering protein and a SNARE protein.
LocusLink. A National Center of Biotechnology Information (NCBI) program that provides a single interface for querying curated sequences and descriptive information about genetic loci., [ http://www.ncbi.nlm.nih.gov/LocusLink/ ]
COILS - prediction of coiled coil regions in proteins. A program for predicting coiled-coil-forming regions, available at the EMBnet website., [ http://www.ch.embnet.org/software/COILS_form.html ]
Download references
Author information
Authors and affiliations.
NCA lab Institute of Molecular and Cell Biology, 30 Medical Drive, Singapore, 117609, Republic of Singapore
Felicia Yu Hsuan Teng, Ya Wang & Bor Luen Tang
Central Imaging and Histology Facility, Institute of Molecular and Cell Biology, 30 Medical Drive, Singapore, 117609, Republic of Singapore
Bor Luen Tang
You can also search for this author in PubMed Google Scholar
Corresponding author
Correspondence to Bor Luen Tang .
Rights and permissions
Reprints and permissions
About this article
Cite this article.
Teng, F.Y.H., Wang, Y. & Tang, B.L. The syntaxins. Genome Biol 2 , reviews3012.1 (2001). https://doi.org/10.1186/gb-2001-2-11-reviews3012
Download citation
Published : 24 October 2001
DOI : https://doi.org/10.1186/gb-2001-2-11-reviews3012
Share this article
Anyone you share the following link with will be able to read this content:
Sorry, a shareable link is not currently available for this article.
Provided by the Springer Nature SharedIt content-sharing initiative
- Vesicular Transport
- Target Membrane
- Snare Complex
- Vesicle Docking
- Early Secretory Pathway
Genome Biology
ISSN: 1474-760X
- Submission enquiries: [email protected]
- General enquiries: [email protected]
Roles of SNARE Proteins in Synaptic Vesicle Fusion
Cite this chapter.
- Mark T. Palfreyman 2 &
- Erik M. Jorgensen 3
Part of the book series: Contemporary Neuroscience ((NEUROBIOL))
1409 Accesses
4 Citations
Neurotransmitters are stored in small membrane-bound vesicles at synapses. Neurotransmitter release is initiated by depolarization of the neuron, which in turn activates voltage-gated calcium channels. Calcium influx then triggers the fusion of the synaptic vesicles with the plasma membrane. Fusion of the vesicular and plasma membranes is mediated by SNARE (soluble N -ethylmaleimide–sensitive factor attachment receptor) proteins. The SNAREs are now known to be used in all trafficking steps of the secretory pathway, including neurotransmission. This chapter describes the discovery of the SNAREs, their relevant structural features, models for their function, the specificity of interactions, and their interactions with the calcium-sensing machinery.
This is a preview of subscription content, log in via an institution to check access.
Access this chapter
- Available as PDF
- Read on any device
- Instant download
- Own it forever
- Available as EPUB and PDF
- Compact, lightweight edition
- Dispatched in 3 to 5 business days
- Free shipping worldwide - see info
Tax calculation will be finalised at checkout
Purchases are for personal use only
Institutional subscriptions
Trimble WS, Cowan DM, Scheller RH. VAMP-1: a synaptic vesicle-associated integral membrane protein. Proc Natl Acad Sci U S A 1988;85(12):4538–4542.
Article CAS PubMed PubMed Central Google Scholar
Inoue A, Obata K, Akagawa K. Cloning and sequence analysis of cDNA for a neuronal cell membrane antigen, HPC-1. J Biol Chem 1992;267(15):10613–10619.
CAS PubMed Google Scholar
Bennett MK, Calakos N, Scheller RH. Syntaxin: a synaptic protein implicated in docking of synaptic vesicles at presynaptic active zones. Science 1992;257(5067):255–259.
Article CAS PubMed Google Scholar
Oyler GA, Higgins GA, Hart RA, et al. The identification of a novel synaptosomal-associated protein, SNAP-25, differentially expressed by neuronal subpopulations. J Cell Biol 1989; 109(6 pt 1):3039–3052.
Brennwald P, Kearns B, Champion K, Keranen S, Bankaitis V, Novick P. Sec9 is a SNAP-25– like component of a yeast SNARE complex that may be the effector of Sec4 function in exocytosis. Cell 1994;79(2):245–258.
Novick P, Field C, Schekman R. Identification of 23 complementation groups required for post-translational events in the yeast secretory pathway. Cell 1980;21(1):205–215.
Burgen AS, Dickens F, Zatman LJ. The action of botulinum toxin on the neuro-muscular junction. J Physiol 1949;109(1–2):10–24.
Blasi J, Chapman ER, Link E, et al. Botulinum neurotoxin A selectively cleaves the synaptic protein SNAP-25. Nature 1993;365(6442):160–163.
Blasi J, Chapman ER, Yamasaki S, Binz T, Niemann H, Jahn R. Botulinum neurotoxin C1 blocks neurotransmitter release by means of cleaving HPC-1/syntaxin. EMBO J 1993;12(12):4821–4828.
CAS PubMed PubMed Central Google Scholar
Link E, Edelmann L, Chou JH, et al. Tetanus toxin action: inhibition of neurotransmitter release linked to synaptobrevin proteolysis. Biochem Biophys Res Commun 1992;189(2): 1017–1023.
Schiavo G, Benfenati F, Poulain B, et al. Tetanus and botulinum-B neurotoxins block neuro-transmitter release by proteolytic cleavage of synaptobrevin. Nature 1992;359(6398):832–835.
Marsal J, Ruiz-Montasell B, Blasi J, et al. Block of transmitter release by botulinum C1 action on syntaxin at the squid giant synapse. Proc Natl Acad Sci U S A 1997;94(26):14871–14876.
O’Connor V, Heuss C, De Bello WM, et al. Disruption of syntaxin-mediated protein interactions blocks neurotransmitter secretion. Proc Natl Acad Sci U S A 1997;94(22):12186–12191.
Article PubMed PubMed Central Google Scholar
Hammarlund M, Palfreyman MT, Watanabe S, Olsen S, Jorgensen EM. Open syntaxin docks synaptic vesicles. PLoS Biol 2007;5(8):e198.
Article PubMed PubMed Central CAS Google Scholar
Broadie K, Prokop A, Bellen HJ, O’Kane CJ, Schulze KL, Sweeney ST. Syntaxin and synapto-brevin function downstream of vesicle docking in Drosophila . Neuron 1995;15(3):663–673.
Schoch S, Deak F, Konigstorfer A, et al. SNARE function analyzed in synaptobrevin/VAMP knockout mice. Science 2001;294(5544):1117–1122.
Washbourne P, Thompson PM, Carta M, et al. Genetic ablation of the t-SNARE SNAP-25 distinguishes mechanisms of neuroexocytosis. Nat Neurosci 2002;5(1):19–26.
Deitcher DL, Ueda A, Stewart BA, Burgess RW, Kidokoro Y, Schwarz TL. Distinct requirements for evoked and spontaneous release of neurotransmitter are revealed by mutations in the Drosophila gene neuronal-synaptobrevin. J Neurosci 1998;18(6):2028–2039.
Vilinsky I, Stewart BA, Drummond JA, Robinson IM, Deitcher DL. A Drosophila SNAP-25 null mutant reveals context-dependent redundancy with SNAP-24 in neurotransmission. Genetics 2002;162(1):259–271.
Balch WE, Glick BS, Rothman JE. Sequential intermediates in the pathway of intercompart-mental transport in a cell-free system. Cell 1984;39(3 Pt 2):525–536.
Wilson DW, Wilcox CA, Flynn GC, et al. A fusion protein required for vesicle-mediated transport in both mammalian cells and yeast. Nature 1989;339(6223):355–359.
Eakle KA, Bernstein M, Emr SD. Characterization of a component of the yeast secretion machinery: identification of the SEC18 gene product. Mol Cell Biol 1988;8(10): 4098–4109.
Block MR, Glick BS, Wilcox CA, Wieland FT, Rothman JE. Purification of an N -ethylmaleimide-sensitive protein catalyzing vesicular transport. Proc Natl Acad Sci U S A 1988;85(21):7852–7856.
Clary DO, Griff IC, Rothman JE. SNAPs, a family of NSF attachment proteins involved in intracellular membrane fusion in animals and yeast. Cell 1990;61(4):709–721.
Bock JB, Matern HT, Peden AA, Scheller RH. A genomic perspective on membrane compartment organization. Nature 2001;409(6822):839–841.
Jahn R, Lang T, Südhof TC. Membrane fusion. Cell 2003;112(4):519–533.
Söllner T, Whiteheart SW, Brunner M, et al. SNAP receptors implicated in vesicle targeting and fusion. Nature 1993;362(6418):318–324.
Article PubMed Google Scholar
Söllner T, Bennett MK, Whiteheart SW, Scheller RH, Rothman JE. A protein assembly-disassembly pathway in vitro may correspond to sequential steps of synaptic vesicle docking, activation, and fusion. Cell 1993;75(3):409–418.
Rothman JE. Intracellular membrane fusion. Adv Second Messenger Phosphoprotein Res 1994;29:81–96.
Mayer A, Wickner W, Haas A. Sec18p (NSF)-driven release of Sec17p (alpha-SNAP) can precede docking and fusion of yeast vacuoles. Cell 1996;85(1):83–94.
Nichols BJ, Ungermann C, Pelham HR, Wickner WT, Haas A. Homotypic vacuolar fusion mediated by t- and v-SNAREs. Nature 1997;387(6629):199–202.
Littleton JT, Chapman ER, Kreber R, Garment MB, Carlson SD, Ganetzky B. Temperature-sensitive paralytic mutations demonstrate that synaptic exocytosis requires SNARE complex assembly and disassembly. Neuron 1998;21(2):401–413.
Grote E, Carr CM, Novick PJ. Ordering the final events in yeast exocytosis. J Cell Biol 2000;151(2):439–452.
Weber T, Zemelman BV, McNew JA, et al. SNAREpins: minimal machinery for membrane fusion. Cell 1998;92(6):759–772.
Hu C, Ahmed M, Melia TJ, Söllner TH, Mayer T, Rothman JE. Fusion of cells by flipped SNAREs. Science 2003;300(5626):1745–1749.
Wickelgren WO, Leonard JP, Grimes MJ, Clark RD. Ultrastructural correlates of transmitter release in presynaptic areas of lamprey reticulospinal axons. J Neurosci 1985;5(5):1188–1201.
Xu-Friedman MA, Harris KM, Regehr WG. Three-dimensional comparison of ultrastructural characteristics at depressing and facilitating synapses onto cerebellar Purkinje cells. J Neurosci 2001;21(17):6666–6672.
Satzler K, Sohl LF, Bollmann JH, et al. Three-dimensional reconstruction of a calyx of Held and its postsynaptic principal neuron in the medial nucleus of the trapezoid body. J Neurosci 2002;22(24):10567–10579.
Schneggenburger R, Meyer AC, Neher E. Released fraction and total size of a pool of immediately available transmitter quanta at a calyx synapse. Neuron 1999;23(2):399–409.
Stevens CF, Tsujimoto T. Estimates for the pool size of releasable quanta at a single central synapse and for the time required to refill the pool. Proc Natl Acad Sci U S A 1995;92(3):846–849.
Rosenmund C, Stevens CF. Definition of the readily releasable pool of vesicles at hippocam-pal synapses. Neuron 1996;16(6):1197–1207.
Fasshauer D, Sutton RB, Brunger AT, Jahn R. Conserved structural features of the synaptic fusion complex: SNARE proteins reclassified as Q- and R-SNAREs. Proc Natl Acad Sci U S A 1998;95(26):15781–15786.
Kloepper TH, Nickias Kienle C, Fasshauer D. An elaborate classification of SNARE proteins sheds light on the conservation of the eukaryotic endomembrane system. Mol Biol Cell 2007;18(9):3463–3471.
Sutton RB, Fasshauer D, Jahn R, Brunger AT. Crystal structure of a SNARE complex involved in synaptic exocytosis at 2.4 A resolution. Nature 1998;395(6700):347–353.
Fasshauer D, Antonin W, Subramaniam V, Jahn R. SNARE assembly and disassembly exhibit a pronounced hysteresis. Nat Struct Biol 2002;9(2):144–151.
Hayashi T, McMahon H, Yamasaki S, et al. Synaptic vesicle membrane fusion complex: action of clostridial neurotoxins on assembly. EMBO J 1994;13(21):5051–5061.
Fratti RA, Collins KM, Hickey CM, Wickner W. Stringent 3Q.1R composition of the SNARE 0–layer can be bypassed for fusion by compensatory SNARE mutation or by lipid bilayer modification. J Biol Chem 2007;282(20):14861–14867.
Ossig R, Schmitt HD, de Groot B, et al. Exocytosis requires asymmetry in the central layer of the SNARE complex. EMBO 2000;19(22):6000–6010.
Article CAS Google Scholar
Wei S, Xu T, Ashery U, et al. Exocytotic mechanism studied by truncated and zero layer mutants of the C-terminus of SNAP-25. EMBO J 2000;19(6):1279–1289.
Wang Y, Dulubova I, Rizo J, Südhof TC. Functional analysis of conserved structural elements in yeast syntaxin Vam3p. J Biol Chem 2001;276(30):28598–28605.
Dilcher M, Kohler B, von Mollard GF. Genetic interactions with the yeast q-SNARE vti1 reveal novel functions for the r-snare ykt6. J Biol Chem 2001;276(37):34537–34544.
Gil A, Gutierrez LM, Carrasco-Serrano C, Alonso MT, Viniegra S, Criado M. Modifications in the C terminus of the synaptosome-associated protein of 25 kDa (SNAP-25) and in the complementary region of synaptobrevin affect the final steps of exocytosis. J Biol Chem 2002;277(12):9904–9910.
Baumert M, Maycox PR, Navone F, De Camilli P, Jahn R. Synaptobrevin: an integral membrane protein of 18,000 daltons present in small synaptic vesicles of rat brain. EMBO J 1989;8(2):379–384.
Parlati F, McNew JA, Fukuda R, Miller R, Söllner TH, Rothman JE. Topological restriction of SNARE-dependent membrane fusion. Nature 2000;407(6801):194–198.
Fiebig KM, Rice LM, Pollock E, Brunger AT. Folding intermediates of SNARE complex assembly. Nat Struct Biol 1999;6(2):117–123.
Fasshauer D, Margittai M. A transient N-terminal interaction of SNAP-25 and syntaxin nucleates SNARE assembly. J Biol Chem 2004;279(9):7613–7621.
Pobbati AV, Stein A, Fasshauer D. N- to C-terminal SNARE complex assembly promotes rapid membrane fusion. Science 2006;313(5787):673–676.
An SJ, Almers W. Tracking SNARE complex formation in live endocrine cells. Science 2004;306(5698):1042–1046.
Fujita Y, Shirataki H, Sakisaka T, et al. Tomosyn: a syntaxin-1–binding protein that forms a novel complex in the neurotransmitter release process. Neuron 1998;20(5):905–915.
Widberg CH, Bryant NJ, Girotti M, Rea S, James DE. Tomosyn interacts with the t-SNAREs syntaxin4 and SNAP23 and plays a role in insulin-stimulated GLUT4 translocation. J Biol Chem 2003;278(37):35093–35101.
Hatsuzawa K, Lang T, Fasshauer D, Bruns D, Jahn R. The R-SNARE motif of tomosyn forms SNARE core complexes with syntaxin 1 and SNAP-25 and down-regulates exocytosis. J Biol Chem 2003;273(33):31159–31166.
McEwen JM, Madison JM, Dybbs M, Kaplan JM. Antagonistic Regulation of Synaptic Vesicle Priming by Tomosyn and UNC-13. Neuron 2006;51(3):303–315.
Gracheva EO, Burdina AO, Holgado AM, et al. Tomosyn inhibits synaptic vesicle priming in Caenorhabditis elegans . PLoS Biol 2006;4(8):e261
Pobbati AV, Razeto A, Boddener M, Becker S, Fasshauer D. Structural basis for the inhibitory role of tomosyn in exocytosis. J Biol Chem 2004;279(45):47192–47200.
Hata Y, Slaughter CA, Südhof TC. Synaptic vesicle fusion complex contains unc-18 homologue bound to syntaxin. Nature 1993;366(6453):347–351.
Garcia EP, Gatti E, Butler M, Burton J, De Camilli P. A rat brain Sec1 homologue related to Rop and UNC18 interacts with syntaxin. Proc Natl Acad Sci U S A 1994;91(6):2003–2007.
Pevsner J, Hsu SC, Scheller RH. n-Sec1: a neural-specific syntaxin-binding protein. Proc Natl Acad Sci U S A 1994;91(4):1445–1449.
Misura KM, Scheller RH, Weis WI. Three-dimensional structure of the neuronal-Sec1–syntaxin 1a complex. Nature 2000;404(6776):355–362.
Gallwitz D, Jahn R. The riddle of the Sec1/Munc-18 proteins—new twists added to their interactions with SNAREs. Trends Biochem Sci 2003;28(3):113–116.
Zilly FE, Sorensen JB, Jahn R, Lang T. Munc18–bound syntaxin readily forms SNARE complexes with synaptobrevin in native plasma membranes. PLoS Biol 2006;4(10):e330.
Shen J, Tareste DC, Paumet F, Rothman JE, Melia TJ. Selective activation of cognate SNAREpins by Sec1/Munc18 proteins. Cell 2007;128(1):183–195.
Rickman C, Medine CN, Bergmann A, Duncan RR. Functionally and spatially distinct modes of MUNC18–syntaxin 1 interaction. J Biol Chem 2007;282(16):12097–12103.
Dulubova I, Khvotchev M, Liu S, Huryeva I, Südhof TC, Rizo J. Munc18–1 binds directly to the neuronal SNARE complex. Proc Natl Acad Sci U S A 2007;104(8):2697–2702.
Liu T, Tucker WC, Bhalla A, Chapman ER, Weisshaar JC. SNARE-driven, 25-millisecond vesicle fusion in vitro . Biophys J 2005;89(4):2458–2472.
Bowen ME, Weninger K, Brunger AT, Chu S. Single molecule observation of liposome-bilayer fusion thermally induced by soluble N -ethyl maleimide sensitive-factor attachment protein receptors (SNAREs). Biophys J 2004;87(5):3569–3584.
Fix M, Melia TJ, Jaiswal JK, et al. Imaging single membrane fusion events mediated by SNARE proteins. Proc Natl Acad Sci U S A 2004;101(19):7311–7316.
Chen YA, Scales SJ, Scheller RH. Sequential SNARE assembly underlies priming and triggering of exocytosis. Neuron 2001;30:161–170.
Hanson PI, Roth R, Morisaki H, Jahn R, Heuser JE. Structure and conformational changes in NSF and its membrane receptor complexes visualized by quick-freeze/deep-etch electron microscopy. Cell 1997;90(3):523–535.
Lin RC, Scheller RH. Structural organization of the synaptic exocytosis core complex. Neuron 1997;19(5):1087–1094.
Zhao C, Slevin JT, Whiteheart SW. Cellular functions of NSF: not just SNAPs and SNAREs. FEBS Lett 2007;581(11):2140–2149.
Marz KE, Lauer JM, Hanson PI. Defining the SNARE complex binding surface of α-SNAP: implications for SNARE complex disassembly. J Biol Chem 2003;278(29):27000–27008.
Scales SJ, Yoo B Y, Scheller RH. The ionic layer is required for efficient dissociation of the SNARE complex by α-SNAP and NSF. Proc Natl Acad Sci U S A 2001;98(25):14262–14267.
Lauer JM, Dalal S, Marz KE, Nonet ML, Hanson PI. SNARE complex zero layer residues are not critical for N -ethylmaleimide-sensitive factor-mediated disassembly. J Biol Chem 2006;281(21):14823–14832.
Langosch D, Hofmann M, Ungermann C. The role of transmembrane domains in membrane fusion. Cell Mol Life Sci 2007;64(7–8):850–864.
Poirier MA, Xiao W, Macosko JC, Chan C, Shin YK, Bennett MK. The synaptic SNARE complex is a parallel four-stranded helical bundle. Nat Struct Biol 1998;5(9):765–769.
Hua S Y, Charlton MP. Activity-dependent changes in partial VAMP complexes during neuro-transmitter release. Nat Neurosci 1999;2(12):1078–1083.
Sorensen JB, Wiederhold K, Muller EM, et al. Sequential N- to C-terminal SNARE complex assembly drives priming and fusion of secretory vesicles. EMBO J 2006;25(5):955–966.
Xu T, Rammner B, Margittai M, Artalejo AR, Neher E, Jahn R. Inhibition of SNARE complex assembly differentially affects kinetic components of exocytosis. Cell 1999;99(7):713–722.
Melia TJ, Weber T, McNew JA, et al. Regulation of membrane fusion by the membrane-proximal coil of the t-SNARE during zippering of SNAREpins. J Cell Biol 2002;158(5):929–940.
Han X, Jackson MB. Structural transitions in the synaptic SNARE complex during Ca2+-triggered exocytosis. J Cell Biol 2006;172(2):281–293.
Chernomordik LV, Kozlov MM. Membrane hemifusion: crossing a chasm in two leaps. Cell 2005;123(3):375–382.
Kasson PM, Kelley NW, Singhal N, Vrljic M, Brunger AT, Pande VS. Ensemble molecular dynamics yields submillisecond kinetics and intermediates of membrane fusion. Proc Natl Acad Sci U S A 2006;103(32):11916–11921.
Cohen FS, Melikyan GB. The energetics of membrane fusion from binding, through hemifu-sion, pore formation, and pore enlargement. J Membr Biol 2004;199(1):1–14.
Kuzmin PI, Zimmerberg J, Chizmadzhev YA, Cohen FS. A quantitative model for membrane fusion based on low-energy intermediates. Proc Natl Acad Sci U S A 2001;98(13): 7235–7240.
Grote E, Baba M, Ohsumi Y, Novick PJ. Geranylgeranylated SNAREs are dominant inhibitors of membrane fusion. J Cell Biol 2000;151(2):453–466.
McNew JA, Weber T, Parlati F, et al. Close is not enough: SNARE-dependent membrane fusion requires an active mechanism that transduces force to membrane anchors. J Cell Biol 2000;150(1):105–117.
Earp LJ, Delos SE, Park HE, White JM. The many mechanisms of viral membrane fusion proteins. Curr Top Microbiol Immunol 2005;285:25–66.
Kozlov MM, Markin VS. [Possible mechanism of membrane fusion]. Biofizika 1983;28(2):242–247.
Knecht V, Mark AE, Marrink SJ. Phase behavior of a phospholipid/fatty acid/water mixture studied in atomic detail. J Am Chem Soc 2006;128(6):2030–2034.
Yang L, Huang HW. Observation of a membrane fusion intermediate structure. Science 2002;297(5588):1877–1879.
Jahn R, Südhof TC. Membrane fusion and exocytosis. Annu Rev Biochem 1999; 68:863–911.
Efrat A, Chernomordik LV, Kozlov MM. Point-like protrusion as a prestalk intermediate in membrane fusion pathway. Biophys J 2007;92(8):L61–63.
Wong JL, Koppel DE, Cowan AE, Wessel GM. Membrane hemifusion is a stable intermediate of exocytosis. Dev Cell 2007;12(4):653–659.
Xu Y, Zhang F, Su Z, McNew JA, Shin YK. Hemifusion in SNARE-mediated membrane fusion. Nat Struct Mol Biol 2005;12(5):417–422.
Lu X, Zhang F, McNew JA, Shin YK. Membrane fusion induced by neuronal SNAREs transits through hemifusion. J Biol Chem 2005;280(34):30538–30541.
Yoon TY, Okumus B, Zhang F, Shin YK, Ha T. Multiple intermediates in SNARE-induced membrane fusion. Proc Natl Acad Sci U S A 2006;103(52):19731–19736.
Zampighi GA, Zampighi LM, Fain N, Lanzavecchia S, Simon SA, Wright EM. Conical electron tomography of a chemical synapse: vesicles docked to the active zone are hemi-fused. Biophys J 2006;91(8):2910–2918.
Melia TJ, You D, Tareste DC, Rothman JE. Lipidic antagonists to SNARE-mediated fusion. J Biol Chem 2006;281(40):29597–29605.
Reese C, Heise F, Mayer A. Trans-SNARE pairing can precede a hemifusion intermediate in intracellular membrane fusion. Nature 2005;436(7049):410–414.
Jun Y, Wickner W. Assays of vacuole fusion resolve the stages of docking, lipid mixing, and content mixing. Proc Natl Acad Sci U S A 2007;104(32):13010–13015.
Kemble GW, Danieli T, White JM. Lipid-anchored influenza hemagglutinin promotes hemi-fusion, not complete fusion. Cell 1994;76(2):383–391.
Chernomordik LV, Zimmerberg J, Kozlov MM. Membranes of the world unite! J Cell Biol 2006;175(2):201–207.
McNew JA, Weber T, Engelman DM, Söllner TH, Rothman JE. The length of the flexible SNAREpin juxtamembrane region is a critical determinant of SNARE-dependent fusion. Mol Cell 1999;4(3):415–421.
Melikyan GB, Brener SA, Ok DC, Cohen FS. Inner but not outer membrane leaflets control the transition from glycosylphosphatidylinositol-anchored influenza hemagglutinin-induced hemifusion to full fusion. J Cell Biol 1997;136(5):995–1005.
Razinkov VI, Melikyan GB, Epand RM, Epand RF, Cohen FS. Effects of spontaneous bilayer curvature on influenza virus-mediated fusion pores. J Gen Physiol 1998;112(4):409–422.
Knecht V, Grubmüller H. Mechanical coupling via the membrane fusion SNARE protein syntaxin 1A: a molecular dynamics study. Biophys J 2003;84(3):1527–1547.
Kiessling V, Tamm LK. Measuring distances in supported bilayers by fluorescence interference-contrast microscopy: polymer supports and SNARE proteins. Biophys J 2003;84(1): 408–418.
Deak F, Shin OH, Kavalali ET, Südhof TC. Structural determinants of synaptobrevin 2 function in synaptic vesicle fusion. J Neurosci 2006;26(25):6668–6676.
Siddiqui TJ, Vites O, Stein A, Heintzmann R, Jahn R, Fasshauer D. Determinants of synap-tobrevin regulation in membranes. Mol Biol Cell 2007;18(6):2037–2046.
Van Komen JS, Bai X, Rodkey TL, Schaub J, McNew JA. The polybasic juxtamembrane region of Sso1p is required for SNARE function in vivo. Eukaryot Cell 2005; 4(12):2017–2028.
Montecucco C, Schiavo G, Pantano S. SNARE complexes and neuroexocytosis: how many, how close? Trends Biochem Sci 2005;30(7):367–372.
Han X, Wang CT, Bai J, Chapman ER, Jackson MB. Transmembrane segments of syntaxin line the fusion pore of Ca2+-triggered exocytosis. Science 2004;304(5668):289–292.
Bowen ME, Engelman DM, Brunger AT. Mutational analysis of synaptobrevin transmembrane domain oligomerization. Biochemistry 2002;41(52):15861–15866.
Roy R, Laage R, Langosch D. Synaptobrevin transmembrane domain dimerization-revisited. Biochemistry 2004;43(17):4964–4970.
Roy R, Peplowska K, Rohde J, Ungermann C, Langosch D. Role of the Vam3p transmembrane segment in homodimerization and SNARE complex formation. Biochemistry 2006;45(24):7654–7660.
Laage R, Rohde J, Brosig B, Langosch D. A conserved membrane-spanning amino acid motif drives homomeric and supports heteromeric assembly of presynaptic SNARE proteins. J Biol Chem 2000;275(23):17481–17487.
Margittai M, Otto H, Jahn R. A stable interaction between syntaxin 1a and synaptobrevin 2 mediated by their transmembrane domains. FEBS Lett 1999;446(1):40–44.
Rickman C, Hu K, Carroll J, Davletov B. Self-assembly of SNARE fusion proteins into star-shaped oligomers. Biochem J 2005;388(1):75–79.
Raciborska DA, Trimble WS, Charlton MP. Presynaptic protein interactions in vivo : evidence from botulinum A, C, D and E action at frog neuromuscular junction. Eur J Neurosci 1998;10(8):2617–2628.
Stewart BA, Mohtashami M, Trimble WS, Boulianne GL. SNARE proteins contribute to calcium cooperativity of synaptic transmission. Proc Natl Acad Sci U S A 2000;97(25): 13955–13960.
Hua Y, Scheller RH. Three SNARE complexes cooperate to mediate membrane fusion. Proc Natl Acad Sci U S A 2001;98(14):8065–8070.
Keller JE, Cai F, Neale EA. Uptake of botulinum neurotoxin into cultured neurons. Biochemistry 2004;43(2):526–532.
Almers W, Tse FW. Transmitter release from synapses: does a preassembled fusion pore initiate exocytosis? Neuron 1990;4(6):813–818.
Ungermann C, Sato K, Wickner W. Defining the functions of trans -SNARE pairs. Nature 1998;396(6711):543–548.
Spruce AE, Breckenridge LJ, Lee AK, Almers W. Properties of the fusion pore that forms during exocytosis of a mast cell secretory vesicle. Neuron 1990;4(5):643–654.
Monck JR, Fernandez JM. The fusion pore and mechanisms of biological membrane fusion. Curr Opin Cell Biol 1996;8(4):524–533.
Peters C, Bayer MJ, Buhler S, Andersen JS, Mann M, Mayer A. Trans -complex formation by proteolipid channels in the terminal phase of membrane fusion. Nature 2001;409 (6820):581–588.
Israel M, Morel N, Lesbats B, Birman S, Manaranche R. Purification of a presynaptic membrane protein that mediates a calcium-dependent translocation of acetylcholine. Proc Natl Acad Sci U S A 1986;83(23):9226–9230.
Peters C, Mayer A. Ca2+/calmodulin signals the completion of docking and triggers a late step of vacuole fusion. Nature 1998;396(6711):575–580.
Hiesinger PR, Fayyazuddin A, Mehta SQ, et al. The v-ATPase V0 subunit a1 is required for a late step in synaptic vesicle exocytosis in Drosophila . Cell 2005;121(4):607–620.
Weber T, Parlati F, McNew JA, et al. SNAREpins are functionally resistant to disruption by NSF and alphaSNAP. J Cell Biol 2000;49(5):1063–1072.
Article Google Scholar
Klyachko VA, Jackson MB. Capacitance steps and fusion pores of small and large-dense-core vesicles in nerve terminals. Nature 2002;418(6893):89–92.
Giaever G, Chu AM, Ni L, et al. Functional profiling of the Saccharomyces cerevisiae genome . Nature 2002;418(6896):387–391.
Chanturiya A, Chernomordik LV, Zimmerberg J. Flickering fusion pores comparable with initial exocytotic pores occur in protein-free phospholipid bilayers. Proc Natl Acad Sci U S A 1997;94(26):14423–14428
Otter-Nilsson M, Hendriks R, Pecheur-Huet E, Hoekstra D, Nilsson T. Cytosolic ATPases, p97 and NSF, are sufficient to mediate rapid membrane fusion. EMBO J 1999;18(8): 2074–2083.
Rizo J, Chen X, Araç D. Unraveling the mechanisms of synaptotagmin and SNARE function in neurotransmitter release. Trends Cell Biol 2006;16(7):339–350.
Brugger B, Nickel W, Weber T, et al. Putative fusogenic activity of NSF is restricted to a lipid mixture whose coalescence is also triggered by other factors. EMBO J 2000;19(6):1272–1278.
Yang B, Gonzalez LJ, Prekeris R, Steegmaier M, Advani RJ, Scheller RH. SNARE interactions are not selective. Implications for membrane fusion specificity. J Biol Chem 1999;274(9):5649–5653.
Tsui MM, Banfield DK. Yeast Golgi SNARE interactions are promiscuous. J Cell Sci 2000;113(1):145–152.
Fasshauer D, Antonin W, Margittai M, Pabst S, Jahn R. Mixed and non-cognate SNARE complexes. Characterization of assembly and biophysical properties. J Biol Chem 1999;274(22):15440–15446.
Parlati F, Varlamov O, Paz K, et al. Distinct SNARE complexes mediating membrane fusion in Golgi transport based on combinatorial specificity. Proc Natl Acad Sci U S A 2002;99(8): 5424–5429.
McNew JA, Parlati F, Fukuda R, et al. Compartmental specificity of cellular membrane fusion encoded in SNARE proteins. Nature 2000;407(6801):153–159.
Scales SJ, Chen YA, Yoo BY, Patel SM, Doung YC, Scheller RH. SNAREs contribute to the specificity of membrane fusion. Neuron 2000;26(2):457–464.
Hunt JM, Bommert K, Charlton MP, et al. A post-docking role for synaptobrevin in synaptic vesicle fusion. Neuron 1994;12(6):1269–1279.
de Wit H, Cornelisse LN, Toonen RF, Verhage M. Docking of secretory vesicles is syntaxin dependent. PLoS ONE 2006;1(1):e126.
Toonen RF, Kochubey O, de Wit H, et al. Dissecting docking and tethering of secretory vesicles at the target membrane. EMBO J 2006;25(16):3725–3737.
Ohara-Imaizumi M, Fujiwara T, Nakamichi Y, et al. Imaging analysis reveals mechanistic differences between first- and second-phase insulin exocytosis. J Cell Biol 2007;177(4):695–705.
Sztul E, Lupashin V. Role of tethering factors in secretory membrane traffic. Am J Physiol Cell Physiol 2006;290(1):C11–26.
Whyte JR, Munro S. Vesicle tethering complexes in membrane traffic. J Cell Sci 2002;115(13):2627–2637.
Zerial M, McBride H. Rab proteins as membrane organizers. Nat Rev Mol Cell Biol 2001;2(2):107–117.
Waters MG, Pfeffer SR. Membrane tethering in intracellular transport. Curr Opin Cell Biol 1999;11(4):453–459.
Guo W, Sacher M, Barrowman J, Ferro-Novick S, Novick P. Protein complexes in transport vesicle targeting. Trends Cell Biol 2000;10(6):251–255.
Wiederkehr A, Du Y, Pypaert M, Ferro-Novick S, Novick P. Sec3p is needed for the spatial regulation of secretion and for the inheritance of the cortical endoplasmic reticulum. Mol Biol Cell 2003;14(12):4770–4782.
Finger FP, Hughes TE, Novick P. Sec3p is a spatial landmark for polarized secretion in budding yeast. Cell 1998;92(4):559–571.
VanRheenen SM, Cao X, Lupashin VV, Barlowe C, Waters MG. Sec35p, a novel peripheral membrane protein, is required for ER to Golgi vesicle docking. J Cell Biol 1998;141(5): 1107–1119.
Wiederkehr A, De Craene JO, Ferro-Novick S, Novick P. Functional specialization within a vesicle tethering complex: bypass of a subset of exocyst deletion mutants by Sec1p or Sec4p. J Cell Biol 2004;167(5):875–887.
Novick P, Medkova M, Dong G, Hutagalung A, Reinisch K, Grosshans B. Interactions between Rabs, tethers, SNAREs and their regulators in exocytosis. Biochem Soc Trans 2006;34(5):683–686.
Borisovska M, Zhao Y, Tsytsyura Y, et al. v-SNAREs control exocytosis of vesicles from priming to fusion. EMBO J 2005;24(12):2114–2126.
Bhattacharya S, Stewart BA, Niemeyer BA, et al. Members of the synaptobrevin/vesicle-associated membrane protein (VAMP) family in Drosophila are functionally interchangeable in vivo for neurotransmitter release and cell viability. Proc Natl Acad Sci U S A 2002;99(21):13867–13872.
Sorensen JB, Nagy G, Varoqueaux F, et al. Differential control of the releasable vesicle pools by SNAP-25 splice variants and SNAP-23. Cell 2003;114(1):75–86.
Holt M, Varoqueaux F, Wiederhold K, et al. Identification of SNAP-47, a novel Qbc-SNARE with ubiquitous expression. J Biol Chem 2006;281(25):17076–17083.
Fujiwara T, Mishima T, Kofuji T, et al. Analysis of knock-out mice to determine the role of HPC-1/syntaxin 1A in expressing synaptic plasticity. J Neurosci 2006;26(21):5767–5776.
Liu Y, Barlowe C. Analysis of Sec22p in endoplasmic reticulum/Golgi transport reveals cellular redundancy in SNARE protein function. Mol Biol Cell 2002;13(9):3314–3324.
Fischer von Mollard G, Stevens TH. The Saccharomyces cerevisiae v-SNARE Vti1p is required for multiple membrane transport pathways to the vacuole. Mol Biol Cell 1999;10 (6):1719–1732.
Darsow T, Rieder SE, Emr SD. A multispecificity syntaxin homologue, Vam3p, essential for autophagic and biosynthetic protein transport to the vacuole. J Cell Biol 1997;138b (11):517–529.
Burgess RW, Deitcher DL, Schwarz TL. The synaptic protein syntaxin1 is required for cel-lularization of Drosophila embryos. J Cell Biol 1997;138(4):861–875.
Betz A, Okamoto M, Benseler F, Brose N. Direct interaction of the rat unc-13 homologue Munc13–1 with the N terminus of syntaxin. J Biol Chem 1997;272(4):2520–2526.
Richmond JE, Weimer RM, Jorgensen EM. An open form of syntaxin bypasses the requirement for UNC-13 in vesicle priming. Nature 2001;412(6844):338–341.
Scales SJ, Hesser BA, Masuda ES, Scheller RH. Amisyn, a novel syntaxin-binding protein that may regulate SNARE complex assembly. J Biol Chem 2002;277(31):28271–28279.
Masuda ES, Huang BC, Fisher JM, Luo Y, Scheller RH. Tomosyn binds t-SNARE proteins via a VAMP-like coiled coil. Neuron 1998;21(3):479–480.
Lao G, Scheuss V, Gerwin CM, et al. Syntaphilin: a syntaxin-1 clamp that controls SNARE assembly. Neuron 2000;25(1):191–201.
Fisher RJ, Pevsner J, Burgoyne RD. Control of fusion pore dynamics during exocytosis by Munc18. Science 2001;291(5505):875–878.
Latham CF, Lopez JA, Hu SH, et al. Molecular dissection of the munc18c/syntaxin4 interaction: implications for regulation of membrane trafficking. Traffic 2006;7(10):1408–1419.
Hu SH, Latham CF, Gee CL, James DE, Martin JL. Structure of the Munc18c/Syntaxin4 N-peptide complex defines universal features of the N-peptide binding mode of Sec1/Munc18 proteins. Proc Natl Acad Sci U S A 2007;104(21):8773–8778.
Togneri J, Cheng YS, Munson M, Hughson FM, Carr CM. Specific SNARE complex binding mode of the Sec1/Munc-18 protein, Sec1p. Proc Natl Acad Sci U S A 2006;103 (47):17730–17735.
Sabatini BL, Regehr WG. Timing of neurotransmission at fast synapses in the mammalian brain. Nature 1996;384(6605):170–172.
Flanagan JJ, Barlowe C. Cysteine-disulfide cross-linking to monitor SNARE complex assembly during endoplasmic reticulum-Golgi transport. J Biol Chem 2006;281 (4):2281–2288.
Starai VJ, Thorngren N, Fratti RA, Wickner W. Ion regulation of homotypic vacuole fusion in Saccharomyces cerevisiae . J Biol Chem 2005;280(17):16754–16762.
McMahon HT, Missler M, Li C, Südhof TC. Complexins: cytosolic proteins that regulate SNAP receptor function. Cell 1995;83(1):111–119.
Perin MS, Fried VA, Mignery GA, Jahn R, Südhof TC. Phospholipid binding by a synaptic vesicle protein homologous to the regulatory region of protein kinase C. Nature 1990;345(6272):260–263.
Giraudo CG, Eng WS, Melia TJ, Rothman JE. A clamping mechanism involved in SNARE-dependent exocytosis. Science 2006;313(5787):676–680.
Tang J, Maximov A, Shin OH, Dai H, Rizo J, Südhof TC. A complexin/synaptotagmin 1 switch controls fast synaptic vesicle exocytosis. Cell 2006;126(6):1175–1187.
Schaub JR, Lu X, Doneske B, Shin YK, McNew JA. Hemifusion arrest by complexin is relieved by Ca2+-synaptotagmin I. Nat Struct Mol Biol 2006;13(8):748–750.
Melia TJ. Putting the clamps on membrane fusion: How complexin sets the stage for calcium-mediated exocytosis. FEBS Lett 2007;581(11):2131–2139.
Bracher A, Kadlec J, Betz H, Weissenhorn W. X-ray structure of a neuronal complexin-SNARE complex from squid. J Biol Chem 2002;277(29):26517–26523.
Chen X, Tomchick DR, Kovrigin E, et al. Three-Dimensional Structure of the Complexin/ SNARE Complex. Neuron 2002;33(3):397–409.
Bai J, Wang CT, Richards DA, Jackson MB, Chapman ER. Fusion Pore Dynamics Are Regulated by Synaptotagmin t-SNARE Interactions. Neuron 2004;41(6):929–942.
Fernandez-Chacon R, Konigstorfer A, Gerber SH, et al. Synaptotagmin I functions as a calcium regulator of release probability. Nature 2001;410(6824):41–49.
Chapman ER. Synaptotagmin: A Ca(2+) sensor that triggers exocytosis? Nat Rev Mol Cell Biol 2002;3(7):498–508.
Davis AF, Bai J, Fasshauer D, Wolowick MJ, Lewis JL, Chapman ER. Kinetics of synapto-tagmin responses to Ca2+ and assembly with the core SNARE complex onto membranes. Neuron 1999;24(4):363–376.
Brose N, Petrenko AG, Südhof TC, Jahn R. Synaptotagmin: a calcium sensor on the synaptic vesicle surface. Science 1992;256(5059):1021–1025.
Bennett MK, Calakos N, Kreiner T, Scheller RH. Synaptic vesicle membrane proteins interact to form a multimeric complex. J Cell Biol 1992;116(3):761–775.
Chapman ER, Hanson PI, An S, Jahn R. Ca2+ regulates the interaction between synaptotagmin and syntaxin 1. J Biol Chem 1995;270(40):23667–23671.
Schiavo G, Stenbeck G, Rothman JE, Söllner TH. Binding of the synaptic vesicle v-SNARE, synaptotagmin, to the plasma membrane t-SNARE, SNAP-25, can explain docked vesicles at neurotoxin-treated synapses. Proc Natl Acad Sci U S A 1997;94(3):997–1001.
Reim K, Mansour M, Varoqueaux F, et al. Complexins regulate a late step in Ca2+-dependent neurotransmitter release. Cell 2001;104(1):71–81.
Tucker WC, Weber T, Chapman ER. Reconstitution of Ca2+-regulated membrane fusion by synaptotagmin and SNAREs. Science 2004;304(5669):435–438.
Mahal LK, Sequeira SM, Gureasko JM, Söllner TH. Calcium-independent stimulation of membrane fusion and SNAREpin formation by synaptotagmin I. J Cell Biol 2002;158 (2):273–283.
Download references
Acknowledgements
We thank Enfu Hui and Edwin R. Chapman for providing versions of Figures 3.1 and 3.3. Thanks also to Winfried Weissenhorn, Dirk Fasshauer, and Reinhard Jahn for allowing us to use and modify their images for Figure 3.1. Michael Ailion, Eric Bend, M. Wayne Davis, and Robert Hobson were instrumental in reading early versions of the manuscript.
Author information
Authors and affiliations.
Department of Biology and Howard Hughes Medical Institute, University of Utah, Salt Lake City, UT, USA
Mark T. Palfreyman
Department of Biology and Howard Hughes Medical Institute, University of Utah, Salt Lake City, UT, 84112, USA
Erik M. Jorgensen
You can also search for this author in PubMed Google Scholar
Corresponding author
Correspondence to Erik M. Jorgensen .
Editor information
Editors and affiliations.
Department of Neuroscience, University of Connecticut Health Center, 263 Farmington Avenue, Farmington, CT, USA
Zhao-Wen Wang Ph.D.
Rights and permissions
Reprints and permissions
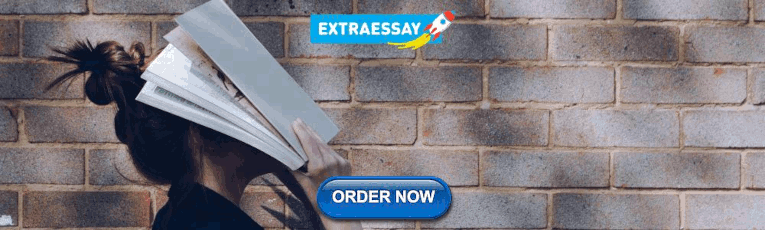
Copyright information
© 2008 Humana Press, a part of Springer Science + Business Media, LLC
About this chapter
Palfreyman, M.T., Jorgensen, E.M. (2008). Roles of SNARE Proteins in Synaptic Vesicle Fusion. In: Wang, ZW. (eds) Molecular Mechanisms of Neurotransmitter Release. Contemporary Neuroscience. Humana Press, Totowa, NJ. https://doi.org/10.1007/978-1-59745-481-0_3
Download citation
DOI : https://doi.org/10.1007/978-1-59745-481-0_3
Publisher Name : Humana Press, Totowa, NJ
Print ISBN : 978-1-934115-38-1
Online ISBN : 978-1-59745-481-0
eBook Packages : Biomedical and Life Sciences Biomedical and Life Sciences (R0)
Share this chapter
Anyone you share the following link with will be able to read this content:
Sorry, a shareable link is not currently available for this article.
Provided by the Springer Nature SharedIt content-sharing initiative
- Publish with us
Policies and ethics
- Find a journal
- Track your research
Thank you for visiting nature.com. You are using a browser version with limited support for CSS. To obtain the best experience, we recommend you use a more up to date browser (or turn off compatibility mode in Internet Explorer). In the meantime, to ensure continued support, we are displaying the site without styles and JavaScript.
- View all journals
- Explore content
- About the journal
- Publish with us
- Sign up for alerts
- News & Views
- Published: July 1998
Probing a complex question: when are SNARE proteins ensnared?
- Timothy A. Ryan 1
Nature Neuroscience volume 1 , pages 175–177 ( 1998 ) Cite this article
287 Accesses
6 Citations
Metrics details
A recent study uses elegant microphysiological and molecular tools to investigate the molecular basis and kinetics of vesicle exocytosis.
This is a preview of subscription content, access via your institution
Access options
Subscribe to this journal
Receive 12 print issues and online access
195,33 € per year
only 16,28 € per issue
Buy this article
- Purchase on Springer Link
- Instant access to full article PDF
Prices may be subject to local taxes which are calculated during checkout
Coloquhoun, D. & Sakmann, B. Neuron 20 , 381–387 (1998).
Article Google Scholar
Xu, T., Binz, T., Niemann, H. & Neher, E. Nature Neurosci. 1 , 192–200 (1998).
Article CAS Google Scholar
Rothman, J.E. Nature 372 , 55–63 (1994).
Scheller, R.H. Neuron 14 , 893–897 (1995).
Söllner, T., Bennett, M.K., Whiteheart, S.W., Scheller, R.H. & Rothman, J.E. Cell 75 , 409–418 (1993).
Weber, T. et al. Cell 92 , 759–772 ( 1998).
Banerjee, A., Barry, V.A., DasGupta, B.R. & Martin, T.F.J. J. Biol. Chem . 271 , 20223–20226 ( 1996).
Schweizer, F.E. et al. Science 279 , 1203–1206 ( 1998).
Hanson, P.I., Roth, R., Morisaki, H., Jahn, R. & Heuser, J.E. Cell 90 , 523–535 (1997).
Fujita, Y . et al. Neuron 20 , 905–915 ( 1998).
Download references
Author information
Authors and affiliations.
Department of Biochemistry, Cornell University Medical College, 1300 York Avenue, New York, 10021, NY, USA
Timothy A. Ryan
You can also search for this author in PubMed Google Scholar
Rights and permissions
Reprints and permissions
About this article
Cite this article.
Ryan, T. Probing a complex question: when are SNARE proteins ensnared?. Nat Neurosci 1 , 175–177 (1998). https://doi.org/10.1038/621
Download citation
Issue Date : July 1998
DOI : https://doi.org/10.1038/621
Share this article
Anyone you share the following link with will be able to read this content:
Sorry, a shareable link is not currently available for this article.
Provided by the Springer Nature SharedIt content-sharing initiative
This article is cited by
Activity-dependent changes in partial vamp complexes during neurotransmitter release.
- Shao-Ying Hua
- Milton P. Charlton
Nature Neuroscience (1999)
Quick links
- Explore articles by subject
- Guide to authors
- Editorial policies
Sign up for the Nature Briefing newsletter — what matters in science, free to your inbox daily.

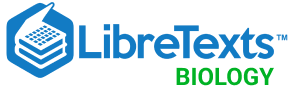
- school Campus Bookshelves
- menu_book Bookshelves
- perm_media Learning Objects
- login Login
- how_to_reg Request Instructor Account
- hub Instructor Commons
- Download Page (PDF)
- Download Full Book (PDF)
- Periodic Table
- Physics Constants
- Scientific Calculator
- Reference & Cite
- Tools expand_more
- Readability
selected template will load here
This action is not available.
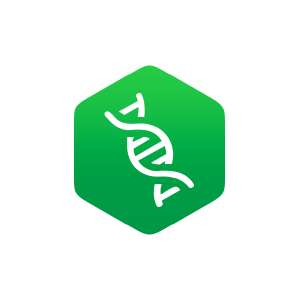
17.5: Directing the Traffic of Proteins in Cells
- Last updated
- Save as PDF
- Page ID 16524
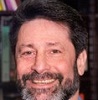
- Gerald Bergtrom
- University of Wisconsin-Milwaukee
Each polypeptide protein translated by ribosomes from a sequence of bases in an mRNA has a specific functional location, either in the cytoplasm, on cellular membranes, inside organelles or in extracellular fluids. In this section we consider the movement and sorting of proteins in the endomembrane system as well as the transport of proteins into and out of organelles
A. Packaging Proteins in the RER
All protein synthesis begins in the same way, with the formation of an initiation complex and subsequent elongation cycles peptide bond formation and carboxylterminal amino acid addition. However, secretory proteins and those destined for lysosomes, peroxisomes or other microbodies, complete elongation directly into cisternae, or spaces enclosed by the rough endoplasmic reticulum ( RER ). It is possible to isolate and purify proteins secreted by cultured cells. A good model system for studying secretory protein synthesis turn out to be mouse myeloma cells.
Mouse myeloma cells were isolated and grown in culture, where they synthesize an IgG light chain , a polypeptide that is part of a mouse immunoglobulin molecule. Immunoglobulins are assembled from light and heavy chain polypeptides and secreted into the circulation. There they serve as circulating antibodies of the vertebrate immune system. Mouse myeloma cells are cancer cells that have lost the ability to make the heavy chain polypeptides. Instead, they secrete mostly the IgG light chain, making it easy to purify it from the cell culture medium. An early experiment revealed that secreted polypeptides made in an in vitro translation system are larger (longer) than the same polypeptides isolated from secretion fluids. This experiment is summarized on the next page.
In one part of the experiment described above, myeloma cells were grown in the presence of radioactive amino acids. The resulting radioactive IgG light chain polypeptides were isolated (follow the red arrows). mRNA separately extracted from another batch of the myeloma cells was added to a cell-free translation system containing radioactive amino acids. The radioactive polypeptide synthesized in vivo and in vitro were separated on electrophoretic gels and autoradiographed (follow the blue arrows, above).
From the autoradiograph, the mature, secreted polypeptides made in vivo had migrated faster on the gel than had those translated in vitro . The cell-free translation product was indeed, larger than the mature secreted polypeptide. To explain these results, Gunther Blobel and colleagues suggested the Signal Hypothesis , according to which secretory protein genes encode extra amino acids as a short amino-terminal signal peptide that directs a growing secretory polypeptide to the RER. To explain the smaller (i.e., shorter) length of the mature, secreted polypeptide, they further proposed that the signal peptide is only a temporary ‘traffic’ signal, removed by an RER-associated enzyme as the polypeptide crossed the RER membrane into the cisternal space.
304 Formulating the Signal Hypothesis: Early Experiments
In the test of the Signal Hypothesis (which won Blobel the 1999 Nobel Prize in Physiology or Medicine), isolated RER membranes were included with mouse myeloma cell mRNA in cell-free protein synthesis systems. Electrophoretic autoradiographs this time showed that the polypeptides made in vitro in the presence of RER were the same size as the mature, secreted polypeptides. The RER must therefore contain processing activity , i.e., a signal peptidase that removes the signal peptide! The steps of the signal hypothesis that emerged from the experiments of Blobel and his colleagues are illustrated below.
Recall that the synthesis of any protein starts with assembly of a translation initiation complex , followed by polypeptide elongation. During elongation, the growing polypeptide moves through and emerges from a channel, or groove in the large subunit. As the N-terminal signal sequence (i.e., the signal peptide) of a secretory polypeptide emerges from this groove, it interacts with the RER membrane. Beginning at the lower left of the illustration above, the steps of the process are:
- An SRP ( signal recognition particle ) binds to the hydrophobic signal peptide .
- Elongation stops until the SRP-ribosome complex finds the RER membrane.
- The ribosome-SRP complex binds to an SRP receptor on the RER membrane.
- The SRP detaches from the growing polypeptide chain, to be recycled.
- Translation elongation resumes through a translocation channel ; a signal peptidase in the RER membrane catalyzes co-translational hydrolysis of the signal peptide, which remains embedded in the RER membrane.
- Elongation continues and the growing polypeptide begins to fold in the RER.
305 Testing the Signal Hypothesis
306 Details of the Signal Hypothesis
Step 2 above requires that the SRP find and bind to the signal peptide before the nascent polypeptide gets too long and starts to fold into a 3D (tertiary) conformation. It turns out the ribosome itself may keep the signal peptide available by destabilizing electrostatic interactions that would otherwise lead to folding and an undoubtedly incorrect conformation. For more on ribosome involvement in protein folding, check out the link at Protein Folding-Destabilizing One Protein Strand at a Time.
The secretory mechanism just described for eukaryotes has its counterpart in bacteria, which secrete proteins that assist in nutrient scavenging as well as cell wall synthesis. Partially elongated signal peptides guide mRNA-bound ribosomes to the cytoplasmic side of the plasma membrane, where the ribosomes bind and then pass elongating proteins through the plasma membrane into the space between the cell membrane and wall. As the protein exits the cell, a bacterial signal peptidase ( SPase ) cleaves the signal peptide. Apparently, the mechanism for the secretion of proteins evolved early and since been conserved. As we will see, this mechanism has been further coopted by eukaryotes for packaging proteins into some organelles and into membranes themselves. Some interesting speculations on the evolution of the protein packaging pathway are discussed in the link below.
307 Destinations of Protein Traffic and Evolution of Pathways
Early on, we discovered that antibiotics stop bacterial growth either by disrupting the cell wall or otherwise killing the cells outright. We now know that some antibiotics (e.g., arylomycins ) disrupt plasma membrane SPase function, preventing proteins required in the space between the cell wall and membrane from ever making it out of the cell. Once used against Staphylococcus aurease , arylomycins are no longer effective because many strains have become resistant to these antibiotics (click Bacterial Signal Peptidase and Antibiotic Resistance to read about the mechanism of arylomycin resistance). As you may already know, S. aurease is now resistant to many antibiotics, and illness from untreatable infections has its own name, MRSA (Methicillin-Resistant Staph Aurease - dig on your own to see more about methicillin resistance). While named for methicillin resistance, MRSA now describes nearly untreatable S. aurease infections.
B. Synthesis of Membrane-Spanning (Integral) Proteins
N-terminal signal sequences also guide ribosomes translating integral membrane proteins to the RER. However, before such a protein can pass completely through the membrane, a stop-transfer sequence (a hydrophobic domain within the polypeptide chain) traps the protein in the fatty acid interior of the membrane. Multiple stoptransfer sequences account for transmembrane proteins that span a membrane more than once (below).
308 Integral Membrane Proteins Hae Stop Transfer Sequences
C. Moving and Sorting Packaged Proteins to Their Final Destination
Like proteins packaged in RER, those made in the cytoplasm go to different destinations before they become functional. Let’s look at the sorting mechanisms for proteins sequestered by the endomembrane system and those made in the cytoplasm.
1. Traffic on the Endomembrane Highway
We have already seen that, once packaged in the RER cisternae, proteins begin post-translational modification (by e.g., ‘core glycosylation’). Transport vesicles that bud off from the RER carry packaged and membrane proteins to the cis vesicles of the Golgi apparatus. There, vesicle fusion is mediated by the recognition of complementary integral membrane proteins embedded in the two membranes. Later, such packaged proteins are sorted to different organelles or to the plasma membrane. Sorting starts as proteins move from the cis to the trans face of the Golgi vesicles, where specific sorting proteins associate with different packaged proteins in the trans Golgi vesicles. The packaged proteins then sort to vesicles that bud off from trans Golgi stacks. These vesicles move to their final destinations, recognizing and fusing with appropriate membranes. Some events of protein trafficking are animated at Events in Protein Trafficking and summarized in the illustration on the next page.
James E. Rothman, Randy W. Schekman and Thomas C. Südhof won the 2013 Nobel Prize in Physiology or Medicine for their studies of the regulation of vesicle traffic (click 2013 Nobel Prize in Physiology or Medicine for more information). Let’s follow some proteins in and on RER membranes through the cell:
- Transition vesicles carrying their mix of packaged proteins bud off from the RER with the help of COPI and COPII coat proteins, and dissociate from the ribosomes originally attached to them. Transition vesicles however, remain associated with the COP proteins.
- These vesicles fuse with the cis Golgi vesicles, a process also mediated by COP proteins. COPI proteins detach during or after fusion, to be recycled back to the RER .
- Packaged proteins and membrane proteins are further processed as the pass through the Golgi vesicle stack, for example undergoing terminal glycosylation.
- At the trans face of the Golgi vesicles, cargo receptor proteins in the membranes to bind specific packaged proteins (now called cargo proteins ). With the help of clathrin and other COP proteins , cargo protein-bound receptor proteins bud off from the trans Golgi stack. However this time, specific cargo proteins sort to separate vesicles with different cellular or extracellular destinations. These budding vesicles also acquire membrane V-SNARE (for vesicle-SNARE) proteins.
- Some vesicles follow this pathway, fusing with lysosomes or similar vesicles to stock them with appropriate enzymes and other protein content. Coat proteins come off the fusing vesicle and are recycled, while vesicle contents are transferred into the next vesicle.
- Vesicles containing secretory proteins typically fuse to form larger secretory vesicles . Secretory vesicles can be stored until the cells are signaled to release their contents from the cell. At that point, secretion vesicles fuse with the plasma membrane, releasing their contents to the extracellular fluid. Once again, coat proteins and clathrin dissociate from the secretory vesicle during fusion.
Other players have been left out of this discussion, notably those that hydrolyze nucleotide triphosphates to provide the energy for this protein trafficking. In addition, you might recognize other molecular players such as clathrin that play a role receptor-mediated endocytosis. Maybe that’s not a surprise! After all, endocytosis is, at least partly, molecular traffic in the opposite direction of vesicle formation and secretion.
2. Nuclear Protein Traffic
Almost all proteins are encoded in the nucleus and translated in the cytosol. These include most of those found in nucleus itself, as well as in mitochondria and chloroplasts (see the Endosymbiotic Hypothesis for a description of intraorganelle gene expression). Proteins synthesized in the cytosol destined for these organelles contain oligopeptide traffic signals that direct them to their appropriate destinations. We saw earlier that large molecules (mRNAs, tRNAs) and even whole particles (i.e., ribosomal subunits) cross the nuclear envelope through nuclear pores.
As for proteins headed for the nucleus, nuclear localization signals rich in positively charged amino acids (lysine, proline) enable binding to the negatively charged domain of a nuclear transport receptor protein in the cytosol. This process is illustrated below.
As the complex of the two proteins approach a nuclear pore, it interacts with nuclear pore fibrils , causing the pore to open. The two bound proteins then cross the double membrane of the nuclear envelope where they accumulate against a concentration gradient. This active transport comes from ATP hydrolysis as the nuclear proteins enter the nucleus.
3. Mitochondrial Protein Traffic
Recall that mitochondria contain their own genome and translational machinery. Thus, they transcribe RNAs and translating proteins of their own. However, genes in the nucleus encode many of the proteins found in mitochondria. Import of these proteins into mitochondria is illustrated below.
Unlike the co-translational packaging of proteins by the RER, mitochondrial protein transfer is post-translational. This means that mitochondrial proteins formed in the cytoplasm have already folded, assuming a tertiary structure. However, the folded protein exposes an N-terminal signal peptide on its surface that recognizes and binds to a receptor protein at the outer mitochondrial membrane. The receptor protein spans both the mitochondrial outer membrane (OM) and cristal membrane (CM).
The receptor protein delivers the protein to membrane contact proteins that also span both mitochondrial membranes. The membrane contact proteins acts as a channel, or pore, through which the mitochondrial protein will cross into the mitochondrial matrix.
But there is a problem: the folded protein cannot cross the membrane by itself! The entry of a completed mitochondrial protein in the cytoplasm requires a so-called chaperone protein, in this case the HSP70 ( heat-shock 70 ) protein. HSP70 controls unfolding of the mitochondrial protein as it passes into the matrix. Upon removal of the signal peptide by a mitochondrial signal peptidase , another HSP70 molecule resident in the mitochondrion facilitates refolding of the protein into a biologically active shape. Recall that HSPs were initially discovered in heat
309 Protein Traffic to Nuclei and How They Communicate
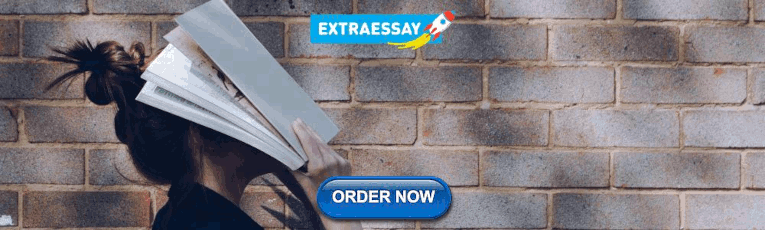
IMAGES
VIDEO
COMMENTS
The SNARE complex. The SNARE hypothesis describes a mechanistic model of membrane fusion based on the characteristics of plasma membranes and exocytosis/neurosecretion. Most of the mechanistic steps necessary for transmitter release occur at the presynaptic region.
The role of SNAREs in docking, proposed by the SNARE hypothesis, ... whereas SNARE pairing is involved at a later step of membrane fusion. Moreover, it is the assembly, ...
Nature Reviews Molecular Cell Biology - SNARE hypothesis 2000. ... Last, the genome of the yeast Saccharomyces cerevisiae does not contain enough SNAREs to specify all the transport steps in the cell.
In one model, assembly of the SNARE complex is stalled at its carboxy-terminal SNARE motifs (at the step in Figure 1 D) by binding to a regulator called complexin. Ca 2+ binding to Syt1 leads to its rapid recruitment to the nearby membranes, increasing the pulling tension between the two fusing membranes.
The SNARE hypothesis holds that a transport vesicle chooses its target for fusion when a soluble NSF attachment protein (SNAP) receptor on the vesicle (v-SNARE) pairs with its cognate t-SNARE at the target membrane. ... A protein assembly-disassembly pathway in vitro that may correspond to sequential steps of synaptic vesicle docking ...
In vitro, SNARE complex formation is a two-step process with a half-time (t 1/2) of 1 min for the first step and a t 1/2 of 1 h for the second step . Without the help of other proteins, complex formation simply cannot support the millisecond fusion observed at the synapse or even the slower events observed in secretory cells.
The Rothman proposal, also called the SNARE hypothesis, ... In an alternative scenario, the docking and fusion particle would form in the usual way as the SNARE complex; however, the final fusion step(s) would be prevented by stabilizing some postdocking-prefusion intermediate, which would be only very briefly present in constitutive ...
The SNARE hypothesis 7 maintains that a SNARE protein on a donor membrane binds to cognate SNAREs on the target membrane in a union (a trans-SNARE complex) that transiently bridges the two ...
During evolution, it was perhaps a small step to couple SNARE-mediated fusion to membrane depolarization, but it was a giant leap for the diversity of life—the nervous system is arguably the universe's greatest invention. ... The first coherent model, called the "SNARE hypothesis," would arise from the melding of the genetic and ...
The role that this phenomenon plays in the fusion process (if any) is unclear, though it is clear that SNARE binding to ion channels can alter the open probability (16) and/or affect the slow inactivation process of the channel (24). Table 1 lists several proteins that have been shown to interact directly with SNAREs.
2 SNARE HYPOTHESIS. SNAREs are a family of 20 to 30 kDa proteins that harbor a conserved SNARE motif of about 60 to 70 residues. ... It was observed that mutations at the C-terminal end of the SNARE bundle led to 2-step unfolding of the SNARE complex in vitro. 80 Analysis of the energetics and dynamics of SNARE complex formation revealed that ...
The first step in vesicle formation is the association of ARF bound to GDP with the Golgi membrane. Proteins in the Golgi membrane then stimulate the exchange of the GDP bound to ARF for GTP, and the COPI coat proteins bind to the ARF/GTP complex. ... called the SNARE hypothesis, in which vesicle fusion is mediated by interactions between ...
The SNARE hypothesis predicts that, as a t-SNARE, the cellular function of a particular syntaxin would logically be determined and restricted by its localization. Thus, it is not surprising to find that syntaxin 18, which is localized to the endoplasmic reticulum (ER), and the cis-Golgi-localized syntaxin 5 have roles in ER-to-Golgi transport ...
The SNARE hypothesis has been proposed to explain both constitutive and regulated vesicular transport in eukaryotic cells, including release of neurotransmitter at synapses. According to this model, a vesicle targeting/docking complex consisting primarily of vesicle- and target-membrane proteins, known as SNAREs, serves as a receptor for the cytosolic N -ethylmaleimide-sensitive fusion protein ...
The SNARE hypothesis Much 18of what we know about SNAREs has ... transport steps, suggesting that a common machinery controls most types of intra-cellular membrane fusion 7,8. A crucial boost
tial step in proceeding from docking to fusion [22]. A group of endoprotease neurotoxins that block neuro- transmitter release in vivo have been found to act by ... The SNARE hypothesis as applied to transport within targeting and fusion, the SNAREs are the best candi-the Golgi stack. Each cisterna is postulated to contain a unique dates to ...
The SNARE hypothesis proposes that membrane traf-ficking specificity is mediated by preferential high af-finity interactions between particular v (vesicle mem- ... at 222 nm (1-min averaging time) in 2 °C steps (2-min equilibration). Data were converted to a fraction of unfolded protein by fitting the lower and upper base lines to 0 and 100% ...
The idea that SNARE proteins mediate vesicle fusion and define trafficking specificity consti-tutes the core of the SNARE hypothesis postulated by Rothman and coworkers (1, 3). Although the role of SNAREs in ves-icle fusion has been generally accepted, the issue of vesicle docking and cargo delivery specificity remains a subject of intense ...
The first coherent model, called the SNARE hypothesis, would arise from the melding of the genetic and biochemical observations described above. ... In yeast, calcium and calmodulin might be required in a step after SNARE complex formation in the process of fusion (139). The target of calcium-calmodulin in this late step in fusion was ...
Membrane fusion is then initiated by calcium-triggering and consists itself of several steps shown in Figures 2B-E. Although the role of SNARE complex formation in mediating exocytosis is widely accepted, the molecular mechanisms underlying the action of SNAREs at individual stages of exocytosis are still debated.
In its original form, the SNARE hypothesis proposed that vesicles would fuse with target membranes via the following sequence of events 3: first, SNAREs on the vesicle and target membranes ...
306 Details of the Signal Hypothesis. Step 2 above requires that the SRP find and bind to the signal peptide before the nascent polypeptide gets too long and starts to fold into a 3D (tertiary) conformation. ... When V-SNARE proteins on their vesicles bind to complementary T-SNARE (for target-SNARE) proteins on receiving membranes, ...
SNARE hypothesis. A proposal for the mechanism by which membranes, particularly vesicular and Golgi or plasma membranes, fuse during, for instance intracellular transport and secretion. The two membranes contain protein complexes, SNAREs, which will become the sites of fusion. The regretable nomenclature derives from an N-ethylmaleimide ...