problem based learning and critical thinking
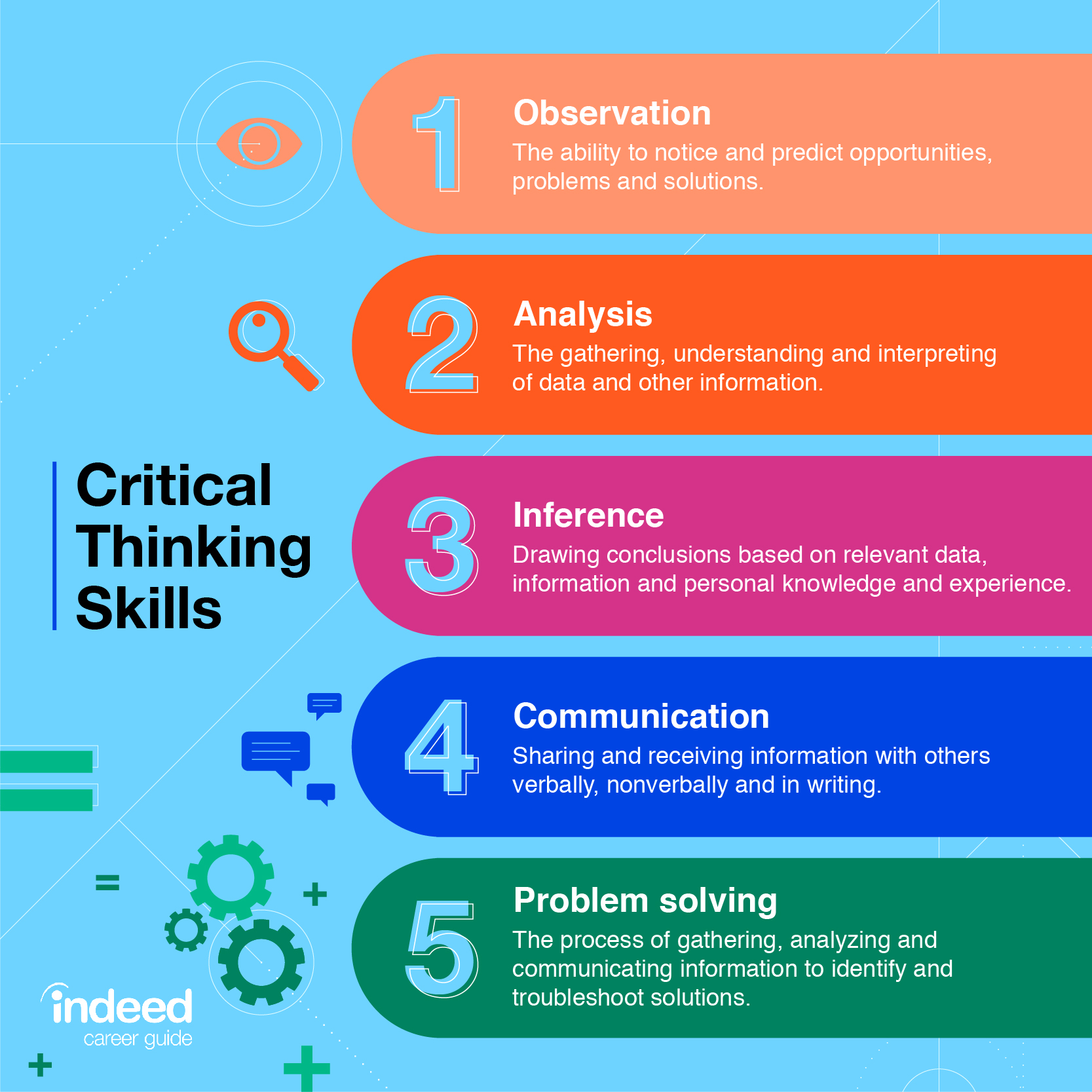
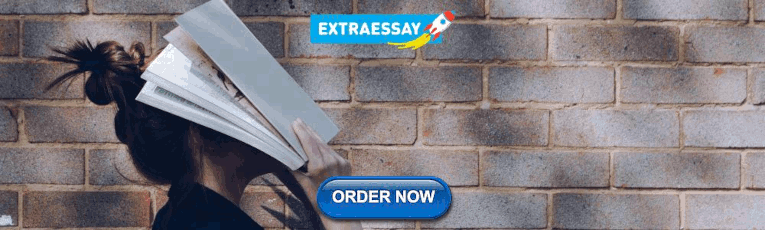
Spatial heterogeneity of soil fertility in coastal zones: a case study of the Yellow River Delta, China
- Soils, Sec 5 • Soil and Landscape Ecology • Research Article
- Published: 25 February 2021
- Volume 21 , pages 1826–1839, ( 2021 )
Cite this article
- Youxiao Wang ORCID: orcid.org/0000-0003-3417-0625 1 , 2 ,
- Gaohuan Liu 1 , 2 &
- Zhonghe Zhao 1 , 2
722 Accesses
12 Citations
Explore all metrics
At the intersection of land and sea, coastal zones are unstable areas that can suffer intensive coastal erosion or sedimentation. The Yellow River Delta, which has experienced rapid land growth, is of great significance in soil fertility research.
Materials and methods
To qualitatively and quantitatively analyse the basic soil fertility characteristics of coastal zones, we sampled six soil chemical indicators obtained from the Yellow River Delta in July 2019. Principal component analysis (PCA) and the Nemoro quality index (NQI) were integrated for the geostatistical analysis of soil fertility by using the ArcGIS and SPSS software.
Results and discussion
The NQI, modified NQI (mNQI) and PCA results significantly represented soil fertility, and the mNQI performed better than the NQI. The soil fertility met the classification of ‘III, generally fertile’. The main factors affecting soil fertility were total potassium, total nitrogen and organic matter. The soil fertility indexes declined consistently from west to east. Increasing distance from the sea significantly negatively affected soil fertility, while soil fertility positively impacted land productivity.
Conclusions
Therefore, future agricultural planning should focus on soil total nitrogen, total potassium and organic matter and control salinization to improve land productivity. The eastern coastal zone, especially the south-eastern region, should be the first area of focus.
This is a preview of subscription content, log in via an institution to check access.
Access this article
Price includes VAT (Russian Federation)
Instant access to the full article PDF.
Rent this article via DeepDyve
Institutional subscriptions
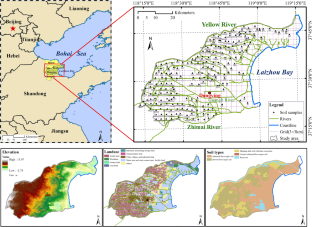
Similar content being viewed by others
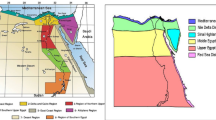
An overview of land degradation, desertification and sustainable land management using GIS and remote sensing applications
Mohamed A. E. AbdelRahman
Pollution indices as useful tools for the comprehensive evaluation of the degree of soil contamination–A review
Joanna Beata Kowalska, Ryszard Mazurek, … Tomasz Zaleski
Soil Salinity: Historical Perspectives and a World Overview of the Problem
Data availability.
All data and calculation tools are available from the author Youxiao Wang ([email protected]).
Andrews SS, Karlen DL, Mitchell JP (2002) A comparison of soil quality indexing methods for vegetable production systems in Northern California. Agric Ecosyst Environ 90:25–45. https://doi.org/10.1016/S0167-8809(01)00174-8
Article Google Scholar
Ashkezari AD, Hosseinzadeh N, Chebli A, Albadi M (2018) Development of an enterprise Geographic Information System (GIS) integrated with smart grid. Sustain Energy, Grids Networks 14:25–34. https://doi.org/10.1016/j.segan.2018.02.001
Bai Y, Mei L, Zuo W, Zhang Y, Gu C, Shan Y, Hu J, Dai Q (2019) Response of bacterial communities in coastal mudflat saline soil to sewage sludge amendment. Appl Soil Ecol 144:107–111. https://doi.org/10.1016/j.apsoil.2019.07.007
Borja A, Bald J, Franco J, Larreta J, Muxika I, Revilla M, Rodríguez JG, Solaun O, Uriarte A, Valencia V (2009) Using multiple ecosystem components, in assessing ecological status in Spanish (Basque Country) Atlantic marine waters. Mar Pollut Bull 59:54–64. https://doi.org/10.1016/j.marpolbul.2008.11.010
Article CAS Google Scholar
Budak M, Gunal H, Celik I et al (2018) Soil quality assesment of upper Tigris basin. Carpathian J Earth Environ Sci 13:301–316. https://doi.org/10.26471/cjees/2018/013/026
Chen Y, Jiang X, Wang Y, Zhuang D (2018) Spatial characteristics of heavy metal pollution and the potential ecological risk of a typical mining area: a case study in China. Process Saf Environ Prot 113:204–219. https://doi.org/10.1016/j.psep.2017.10.008
Chen S, Lin B, Li Y, Zhou S (2020) Spatial and temporal changes of soil properties and soil fertility evaluation in a large grain-production area of subtropical plain, China. Geoderma 357:113937. https://doi.org/10.1016/j.geoderma.2019.113937
Cullimore DR (1966) A qualitative method of assessing the available nitrogen, potassium and phosphorus in the soil. J Sci Food Agric 17:321–323. https://doi.org/10.1002/jsfa.2740170709
Delgado-Baquerizo M, Powell JR, Hamonts K, Reith F, Mele P, Brown MV, Dennis PG, Ferrari BC, Fitzgerald A, Young A, Singh BK, Bissett A (2017) Circular linkages between soil biodiversity, fertility and plant productivity are limited to topsoil at the continental scale. New Phytol 215:1186–1196. https://doi.org/10.1111/nph.14634
Elidrissi S, Omdi FE, El Azhari A et al (2020) New application of GIS and statistical analysis in mapping the distribution of quaternary calcrete (Tensift Al Haouz area, Central Morocco). Catena 188:104419. https://doi.org/10.1016/j.catena.2019.104419
Fan X, Liu G, Tang Z, Shu L (2010) Analysis on main contributors influencing soil salinization of Yellow River Delta. J Soil Water Conserv 24:139–144. https://doi.org/10.13870/j.cnki.stbcxb.2010.01.030
Fang J, Lincke D, Brown S, Nicholls RJ, Wolff C, Merkens JL, Hinkel J, Vafeidis AT, Shi P, Liu M (2020) Coastal flood risks in China through the 21st century–an application of DIVA. Sci Total Environ 704:135311. https://doi.org/10.1016/j.scitotenv.2019.135311
Fayiah M, Dong S, Li Y, Xu Y, Gao X, Li S, Shen H, Xiao J, Yang Y, Wessell K (2019a) The relationships between plant diversity, plant cover, plant biomass and soil fertility vary with grassland type on Qinghai-Tibetan Plateau. Agric Ecosyst Environ 286:106659. https://doi.org/10.1016/j.agee.2019.106659
Fayiah M, Dong S, Li Y, Xu Y, Gao X, Li S, Shen H, Xiao J, Yang Y, Wessell K (2019b) The relationships between plant diversity, plant cover, plant biomass and soil fertility vary with grassland type on Qinghai-Tibetan Plateau. Agric Ecosyst Environ 286:106659. https://doi.org/10.1016/j.agee.2019.106659
Fei X, Xiao R, Christakos G, Langousis A, Ren Z, Tian Y, Lv X (2019) Comprehensive assessment and source apportionment of heavy metals in Shanghai agricultural soils with different fertility levels. Ecol Indic 106:105508. https://doi.org/10.1016/j.ecolind.2019.105508
Fotheringham AS, Charlton ME, Brunsdon C (1998) Geographically weighted regression: a natural evolution of the expansion method for spatial data analysis. Environ Plan A 30:1905–1927. https://doi.org/10.1068/a301905
Fu J, Jiang D, Huang Y (2014) China km grid population distribution dataset. Acta Geograph Sin 69:41–44. https://doi.org/10.11821/dlxb2014S006
Guo L, Sun Z, Ouyang Z, Han D, Li F (2017) A comparison of soil quality evaluation methods for Fluvisol along the lower Yellow River. Catena 152:135–143. https://doi.org/10.1016/j.catena.2017.01.015
Hamidi Nehrani S, Askari MS, Saadat S, Delavar MA, Taheri M, Holden NM (2020) Quantification of soil quality under semi-arid agriculture in the northwest of Iran. Ecol Indic 108:105770. https://doi.org/10.1016/j.ecolind.2019.105770
He H, Miao Y, Gan Y, Wei S, Tan S, Rask KA, Wang L, Dai J, Chen W, Ekelund F (2020) Soil bacterial community response to long-term land use conversion in Yellow River Delta. Appl Soil Ecol 156:103709. https://doi.org/10.1016/j.apsoil.2020.103709
Hu M, Huang Y (2020) Atakrig: an R package for multivariate area-to-area and area-to-point kriging predictions. Comput Geosci 139:104471. https://doi.org/10.1016/j.cageo.2020.104471
Huang Y, Jiang D, Fu J (2014) China km grid GDP distribution dataset. Acta Geograph Sin 69:45–48. https://doi.org/10.11821/dlxb2014S007
Janssen BH, de Willigen P (2006) Ideal and saturated soil fertility as bench marks in nutrient management. 1. Outline of the framework. Agriculture. Ecosyst Environ 116:132–146. https://doi.org/10.1016/j.agee.2006.03.014
Ji H, Pan S, Chen S (2020) Impact of river discharge on hydrodynamics and sedimentary processes at Yellow River Delta. Mar Geol 425:106210. https://doi.org/10.1016/j.margeo.2020.106210
Jiang M, Xu L, Chen X, Zhu H, Fan H (2020) Soil quality assessment based on a minimum data set: a case study of a county in the typical river delta wetlands. Sustainability (Switzerland) 12:1–21. https://doi.org/10.3390/su12219033
Jiao S, Li J, Li Y, Xu Z, Kong B, Li Y, Shen Y (2020) Variation of soil organic carbon and physical properties in relation to land uses in the Yellow River Delta, China. Sci Rep 10:1–12. https://doi.org/10.1038/s41598-020-77303-8
Jin G, Fang W, Shafi M, Wu D, Li Y, Zhong B, Ma J, Liu D (2019) Source apportionment of heavy metals in farmland soil with application of APCS-MLR model: a pilot study for restoration of farmland in Shaoxing City Zhejiang, China. Ecotoxicol Environ Saf 184:109495. https://doi.org/10.1016/j.ecoenv.2019.109495
Kan W, Wu Q (1994) Preliminary study on a method for quantitative comprehensive evaluation of soil fertility. Chin J Soil Sci 245-247. https://doi.org/10.19336/j.cnki.trtb.1994.06.002
Levi N, Karnieli A, Paz-Kagan T (2020) Using reflectance spectroscopy for detecting land-use effects on soil quality in drylands. Soil Tillage Res 199:104571. https://doi.org/10.1016/j.still.2020.104571
Li P, Zhang T, Wang X, Yu D (2013) Development of biological soil quality indicator system for subtropical China. Soil Tillage Res 126:112–118. https://doi.org/10.1016/j.still.2012.07.011
Li J, Pu L, Zhu M, Zhang J, Li P, Dai X, Xu Y, Liu L (2014) Evolution of soil properties following reclamation in coastal areas: a review. Geoderma 226–227:130–139. https://doi.org/10.1016/j.geoderma.2014.02.003
Li S, Lei Y, Zhang Y, Liu J, Shi X, Jia H, Wang C, Chen F, Chu Q (2019a) Rational trade-offs between yield increase and fertilizer inputs are essential for sustainable intensification: a case study in wheat–maize cropping systems in China. Sci Total Environ 679:328–336. https://doi.org/10.1016/j.scitotenv.2019.05.085
Li X, Wang D, Ren Y, Wang Z, Zhou Y (2019b) Soil quality assessment of croplands in the black soil zone of Jilin Province, China: establishing a minimum data set model. Ecol Indic 107:105251. https://doi.org/10.1016/j.ecolind.2019.03.028
Liu Z, Lu B, Xiao H, Liu D, Li X, Wang LA, Urbanovich O, Nagorskaya L (2019) Effect of mixed solutions of heavy metal eluents on soil fertility and microorganisms. Environ Pollut 254:254. https://doi.org/10.1016/j.envpol.2019.112968
Luo X, Liu G, Xia Y, Chen L, Jiang Z, Zheng H, Wang Z (2017) Use of biochar-compost to improve properties and productivity of the degraded coastal soil in the Yellow River Delta, China. J Soils Sediments 17:780–789. https://doi.org/10.1007/s11368-016-1361-1
Luo J, Zhang X, Wu Y, Shen J, Shen L, Xing X (2018) Urban land expansion and the floating population in China: for production or for living? Cities 74:219–228. https://doi.org/10.1016/j.cities.2017.12.007
Mahmoody Vanolya N, Jelokhani-Niaraki M, Toomanian A (2019) Validation of spatial multicriteria decision analysis results using public participation GIS. Appl Geogr 112:102061. https://doi.org/10.1016/j.apgeog.2019.102061
Mi W, Sun Y, Xia S, Zhao H, Mi W, Brookes PC, Liu Y, Wu L (2018) Effect of inorganic fertilizers with organic amendments on soil chemical properties and rice yield in a low-productivity paddy soil. Geoderma 320:23–29. https://doi.org/10.1016/j.geoderma.2018.01.016
Nabiollahi K, Golmohamadi F, Taghizadeh-Mehrjardi R, Kerry R, Davari M (2018) Assessing the effects of slope gradient and land use change on soil quality degradation through digital mapping of soil quality indices and soil loss rate. Geoderma 318:16–28. https://doi.org/10.1016/j.geoderma.2017.12.024
National Soil Census Office (1998) Chinese soil, 1st edn. China Agricultural Press, Beijing
Google Scholar
Ottinger M, Kuenzer C, Liu G, Wang S, Dech S (2013) Monitoring land cover dynamics in the Yellow River Delta from 1995 to 2010 based on Landsat 5 TM. Appl Geogr 44:53–68. https://doi.org/10.1016/j.apgeog.2013.07.003
Panico SC, Esposito F, Memoli V, Vitale L, Polimeno F, Magliulo V, Maisto G, de Marco A (2020) Variations of agricultural soil quality during the growth stages of sorghum and sunflower. Appl Soil Ecol 152:152. https://doi.org/10.1016/j.apsoil.2020.103569
Patzel N, Sticher H, Karlen DL (2000) Soil fertility-phenomenon and concept. J Plant Nutr Soil Sci 163:129–142. https://doi.org/10.1002/(SICI)1522-2624(200004)163:2<129::AID-JPLN129>3.0.CO;2-D
Peigné J, Vian JF, Payet V, Saby NPA (2018) Soil fertility after 10 years of conservation tillage in organic farming. Soil Tillage Res 175:194–204. https://doi.org/10.1016/j.still.2017.09.008
Qi Y, Darilek JL, Huang B, Zhao Y, Sun W, Gu Z (2009) Evaluating soil quality indices in an agricultural region of Jiangsu Province, China. Geoderma 149:325–334. https://doi.org/10.1016/j.geoderma.2008.12.015
Santos-Francés F, Martínez-Graña A, Ávila-Zarza C, Criado M, Sánchez Y (2019) Comparison of methods for evaluating soil quality of semiarid ecosystem and evaluation of the effects of physico-chemical properties and factor soil erodibility (Northern Plateau, Spain). Geoderma 354:113872. https://doi.org/10.1016/j.geoderma.2019.07.030
Sun H, Jin Q, Wang Q, Shao C, Zhang L, Guan Y, Tian H, Li M, Zhang Y (2020) Effects of soil quality on effective ingredients of Astragalus mongholicus from the main cultivation regions in China. Ecol Indic 114:106296. https://doi.org/10.1016/j.ecolind.2020.106296
Tang L, Hayashi K, Ohigashi K, Shimura M, Kohyama K (2019) Developing characterization factors to quantify management impacts on soil quality of paddy fields within life cycle assessment. J Clean Prod 238:117890. https://doi.org/10.1016/j.jclepro.2019.117890
Tresch S, Moretti M, Le Bayon RC et al (2018) Urban soil quality assessment-a comprehensive case study dataset of urban garden soils. Front Environ Sci 6:1–5. https://doi.org/10.3389/fenvs.2018.00136
Valiente R, Escobar F, Pearce J, Bilal U, Franco M, Sureda X (2020) Estimating and mapping cigarette butt littering in urban environments: a GIS approach. Environ Res 183:109142. https://doi.org/10.1016/j.envres.2020.109142
Vasu D, Singh SK, Ray SK, Duraisami VP, Tiwary P, Chandran P, Nimkar AM, Anantwar SG (2016) Soil quality index (SQI) as a tool to evaluate crop productivity in semi-arid Deccan plateau, India. Geoderma 282:70–79. https://doi.org/10.1016/j.geoderma.2016.07.010
Vrščaj B, Poggio L, Marsan FA (2008) A method for soil environmental quality evaluation for management and planning in urban areas. Landsc Urban Plan 88:81–94. https://doi.org/10.1016/j.landurbplan.2008.08.005
Wang H, Yao L, Huang B et al (2019a) An integrated approach to exploring soil fertility from the perspective of rice (Oryza sativa L.) yields. Soil Tillage Res. https://doi.org/10.1016/j.still.2019.104322
Wang H, Yao L, Huang B et al (2019b) An integrated approach to exploring soil fertility from the perspective of rice (Oryza sativa L.) yields. Soil Tillage Res. https://doi.org/10.1016/j.still.2019.104322
Wang X, Guo T, Wang Y, Xing Y, Wang Y, He X (2020) Exploring the optimization of water and fertilizer management practices for potato production in the sandy loam soils of Northwest China based on PCA. Agric Water Manag 237:106180. https://doi.org/10.1016/j.agwat.2020.106180
Wu D (2020) Spatially and temporally varying relationships between ecological footprint and influencing factors in China’s provinces using Geographically Weighted Regression (GWR). J Clean Prod 261:121089. https://doi.org/10.1016/j.jclepro.2020.121089
Wu C, Liu G, Huang C, Liu Q (2019) Soil quality assessment in Yellow River Delta: establishing a minimum data set and fuzzy logic model. Geoderma 334:82–89. https://doi.org/10.1016/j.geoderma.2018.07.045
Xia M, Zhao B, Hao X, Zhang J (2015) Soil quality in relation to agricultural production in the North China Plain. Pedosphere 25:592–604. https://doi.org/10.1016/S1002-0160(15)30039-4
Xu X, Chen Z, Feng Z (2019) From natural driving to artificial intervention: changes of the Yellow River estuary and delta development. Ocean Coast Manag 174:63–70. https://doi.org/10.1016/j.ocecoaman.2019.03.009
Yageta Y, Osbahr H, Morimoto Y, Clark J (2019) Comparing farmers’ qualitative evaluation of soil fertility with quantitative soil fertility indicators in Kitui County, Kenya. Geoderma 344:153–163. https://doi.org/10.1016/j.geoderma.2019.01.019
Yang Y, Tong X, Ruan H (2012) Comprehensive evaluation of soil fertility quality based on field data. Transact Chin Soc Agric Eng 28:151–156. https://doi.org/10.3969/j.issn.1002-6819.2012.z2.026
Yuan Y, Cave M, Xu H, Zhang C (2020) Exploration of spatially varying relationships between Pb and Al in urban soils of London at the regional scale using geographically weighted regression (GWR). J Hazard Mater 393:122377. https://doi.org/10.1016/j.jhazmat.2020.122377
Zeraatpisheh M, Bakhshandeh E, Hosseini M, Alavi SM (2020) Assessing the effects of deforestation and intensive agriculture on the soil quality through digital soil mapping. Geoderma 363:114139. https://doi.org/10.1016/j.geoderma.2019.114139
Zhang X, Zhu A, Xin X, Yang W, Zhang J, Ding S (2018) Tillage and residue management for long-term wheat-maize cropping in the North China Plain: I. Crop yield and integrated soil fertility index. Field Crop Res 221:157–165. https://doi.org/10.1016/j.fcr.2018.02.025
Zhang D, Shi X, Xu H et al (2020) A GIS-based spatial multi-index model for flood risk assessment in the Yangtze River Basin, China. Environ Impact Assess Rev. https://doi.org/10.1016/j.eiar.2020.106397
Zheng R, Zhao J, Zhou X et al (2016) Land use effects on the distribution and speciation of heavy metals and arsenic in coastal soils on Chongming Island in the Yangtze River Estuary, China. Pedosphere 26:74–84. https://doi.org/10.1016/S1002-0160(15)60024-8
Download references
This research was supported by the National Key R&D Program of China, Grant No. 2017YFD0300403; the Strategic Priority Research Program of Chinese Academy of Sciences, Grant No. XDA23050101; and the National Natural Science Foundation of China, Grant No. 42001227.
Author information
Authors and affiliations.
State Key Laboratory of Resources and Environmental Information System, Institute of Geographic Sciences and Natural Resources Research, Chinese Academy of Sciences, Beijing, 100101, China
Youxiao Wang, Gaohuan Liu & Zhonghe Zhao
University of Chinese Academy of Sciences, Beijing, 100049, China
You can also search for this author in PubMed Google Scholar
Corresponding author
Correspondence to Gaohuan Liu .
Ethics declarations
Conflict of interest.
The authors declare no competing interests.
Additional information
Responsible editor: Jun Zhou
The work described is an original research that has not been published previously and is not under consideration for publication elsewhere in whole or in part.
Publisher’s note
Springer Nature remains neutral with regard to jurisdictional claims in published maps and institutional affiliations.
(PNG 1789 kb)
High Resolution Image (TIF 2426 kb)
(PNG 463 kb)
High Resolution Image (TIF 702 kb)
(PNG 78 kb)
High Resolution Image (TIF 482 kb)
(PNG 4155 kb)
High Resolution Image (TIF 4921 kb)
(PNG 3849 kb)
High Resolution Image (TIF 5025 kb)
Rights and permissions.
Reprints and permissions
About this article
Wang, Y., Liu, G. & Zhao, Z. Spatial heterogeneity of soil fertility in coastal zones: a case study of the Yellow River Delta, China. J Soils Sediments 21 , 1826–1839 (2021). https://doi.org/10.1007/s11368-021-02891-5
Download citation
Received : 30 August 2020
Accepted : 25 January 2021
Published : 25 February 2021
Issue Date : April 2021
DOI : https://doi.org/10.1007/s11368-021-02891-5
Share this article
Anyone you share the following link with will be able to read this content:
Sorry, a shareable link is not currently available for this article.
Provided by the Springer Nature SharedIt content-sharing initiative
- Yellow River Delta
- Soil fertility index
- Principal component analysis
- Nemoro quality index
- Geostatistical analysis
- Find a journal
- Publish with us
- Track your research
Accessibility Links
- Skip to content
- Skip to search IOPscience
- Skip to Journals list
- Accessibility help
- Accessibility Help
Click here to close this panel.

Purpose-led Publishing is a coalition of three not-for-profit publishers in the field of physical sciences: AIP Publishing, the American Physical Society and IOP Publishing.
Together, as publishers that will always put purpose above profit, we have defined a set of industry standards that underpin high-quality, ethical scholarly communications.
We are proudly declaring that science is our only shareholder.
Prevention and control measures for coastal erosion in northern high-latitude communities: a systematic review based on Alaskan case studies
Min Liew 1,4 , Ming Xiao 1 , Benjamin M Jones 2 , Louise M Farquharson 3 and Vladimir E Romanovsky 3
Published 19 August 2020 • © 2020 The Author(s). Published by IOP Publishing Ltd Environmental Research Letters , Volume 15 , Number 9 Citation Min Liew et al 2020 Environ. Res. Lett. 15 093002 DOI 10.1088/1748-9326/ab9387
You need an eReader or compatible software to experience the benefits of the ePub3 file format .
Article metrics
17642 Total downloads 139 Video abstract views
Share this article
Author e-mails.
Author affiliations
1 Department of Civil and Environmental Engineering, The Pennsylvania State University, University Park, PA 16802, United States of America
2 Institute of Northern Engineering, University of Alaska Fairbanks, Fairbanks, AK 99775, United States of America
3 Geophysical Institute, University of Alaska Fairbanks, Fairbanks, AK 99775, United States of America
4 Author to whom any correspondence should be addressed.
Min Liew https://orcid.org/0000-0002-5156-4610
Benjamin M Jones https://orcid.org/0000-0002-1517-4711
- Received 13 November 2019
- Accepted 15 May 2020
- Published 19 August 2020
Peer review information
Method : Single-anonymous Revisions: 1 Screened for originality? Yes
Buy this article in print
Erosion along high-latitude coasts has been accelerating in recent decades, resulting in land loss and infrastructure damage, threatening the wellbeing of local communities, and forcing undesired community relocations. This review paper evaluates the state of practice of current coastal stabilization measures across several coastal communities in northern high latitudes. After considering global practices and those in northern high latitude and arctic settings, this paper then explores new and potential coastal stabilization measures to address erosion specific to northern high-latitude coastlines. The challenges in constructing the current erosion control measures and the cost of the measures over the last four decades in northern high-latitude regions are presented through case histories. The synthesis shows that among the current erosion controls being used at high latitudes, revetments built with rocks have the least reported failures and are the most common measures applied along northern high-latitude coastlines including permafrost coasts, while riprap is the most common material used. For seawalls, bulkheads, and groin systems, reported failures are common and mostly associated with displacement, deflection, settlement, vandalism, and material ruptures. Revetments have been successfully implemented at sites with a wide range of mean annual erosion rates (0.3–2.4 m/year) and episodic erosion (6.0–22.9 m) due to the low costs and easy construction, inspection, and decommissioning. No successful case history has been reported for the non-engineered expedient measures that are constructed in the event of an emergency, except for the expedient vegetation measure using root-wads and willows. Soft erosion prevention measures, which include both beach nourishment and dynamically stable beaches, have been considered in this review. The effectiveness of beach nourishment in Utqiaġvik, Alaska, which is affected by permafrost, is inconclusive. Dynamically stable beaches are effective in preventing erosion, and observations show that they experience only minor damages after single storm events. The analysis also shows that more measures have been constructed on a spit (relative to bluffs, islands, barrier islands, and river mouths), which is a landform where many Alaskan coastal communities reside. The emerging erosion control measures that can potentially be adapted to mitigate coastal erosion in high-latitude regions include geosynthetics, static bay beach concept, refrigerating techniques, and biogeochemical applications. However, this review shows that there is a lack of case studies that evaluated the performance of these new measures in high-latitude environments. This paper identifies research gaps so that these emerging measures can be upscaled for full-scale applications on permafrost coasts.
Export citation and abstract BibTeX RIS
1. Introduction
High-latitude coastlines are influenced by several factors that set them apart from lower latitude coastlines including the presence of sea-ice and permafrost and are among the most dynamic in the world (Jones et al 2009a , Mars and Houseknecht 2007 , Overeem et al 2011 , Lantuit et al 2012 , Farquharson et al 2018 , Irrgang et al 2018 , Novikova et al 2018 In some cases, high rates of coastal change can threaten coastal infrastructure and communities and create a need for erosion prevention. Solomon and Covill ( 1995 ) and Cunliffe et al ( 2019 ) noted that, rather than a slow steady process, erosion can occur in an episodic manner with short-term erosion rates that greatly exceed the long-term average rate. Accelerating rates of erosion at high-latitudes can be attributed to a longer open-water period or sea ice decline (Overeem et al 2011 , Cai et al 2018 , Farquharson et al 2018 , Kwok 2018 ), shifting of shorefast ice to frazil ice (Aré et al 2008 ), shifting of multiyear ice to seasonal sea ice (Kwok 2018 ), sea surface temperature (Costard et al 2007 , Overeem et al 2011 ), more frequent or severe storms (Manson and Solomon 2007 ), and the warming and thawing of permafrost (Nelson et al 2001 , Romanovsky et al 2010 , Rowland et al 2010 , Grosse et al 2011 , Sinitsyn et al 2020 ). More recently, Jones et al ( 2018 ) demonstrated a sustained increase in erosion over the last decade along the highly dynamic permafrost-affected Drew Point section of the Beaufort Sea coastline and the complexities associated with the factors most responsible for interannual variability in permafrost bluff erosion. Farquharson et al ( 2018 ) also demonstrated the complexities of coastal changes in permafrost regions by highlighting the high spatial variability of these changes across various types of coastal morphology and an increasingly dynamic permafrost coast of northwest Alaska. Lantuit and Pollard ( 2008 ) also reported such complexities after observing a decrease in mean annual rate of shoreline erosion on Herschel Island, which is also a permafrost-affected region, but an increase in erosion rate for segments of these shorelines with high ground ice content.
The economic impacts of climate change on Alaska's infrastructure and land losses associated with coastal erosion have been quantified under various climate forcing scenarios (Cole et al 1999 , Larsen et al 2008 , Melvin et al 2016 ). In general, studies indicate that projected climate change could impose additional costs due to infrastructure damage in Alaska and across the pan-Arctic (Hinkel et al 2003 , Hjort et al 2018 ). Such climate-induced expenditures increase substantially as more comprehensive infrastructure inventories are considered. The uncertainties in climate projections (Friedlingstein et al 2014 , Nordhaus 2018 ) may also increase the uncertainties in these predicted expenditures. A recent study by Melvin et al ( 2016 ) showed that cumulative costs of climate-related damages to Alaskan infrastructure from year 2015 to 2099 were estimated to be $5.5 billion for Representative Concentration Pathway (RCP) 8.5 (representing the highest greenhouse gas emissions scenario projected by the Intergovernmental Panel on Climate Change (IPCC)) and $4.2 billion for RCP4.5 (representing stabilizing greenhouse gas emissions scenario).
Due to the severe impacts of coastal erosion on the lives and livelihood of northern indigenous communities and the high costs of implementing coastal protection, some villages have started to consider community-based relocation. The urgency and constraints of community-based relocations due to coastal erosion have been discussed in many studies (Shearer 2012 , Marino 2012 , Bronen and Chapin 2013 , Gorokhovich et al 2013 , Maldonado et al 2013 , Bronen 2015 ). These studies generally agree that erosion, which has been considered a slow geomorphological process, should now be included under the statutory definition of disaster, and relocations should be community-led and government-supported so that the displacement efforts are in agreement with the culture and traditional values of local communities (Bronen et al 2019 ).
In some northern high-latitude populated regions with high coastal erosion rates, plans were established for community relocations and efforts are underway for the construction of erosion control structures (Radosavljevic et al 2016 , Novikova et al 2018 , Irrgang et al 2019 ). The efforts, however, are insufficient to address coastal erosion in high-latitude regions, especially those influenced by permafrost, and are currently of little help to local residents in adapting to and transitioning into a new coastal norm that is characterized by high erosion rates and a more dynamic coastal system. This is because the erosion prevention structures were designed based on their local historical trends, while the trends are dynamically changing and the structures can no longer be effective. Given the social context of the indigenous people and their long history of ties to the land and sea, community-based relocations are often complex and not feasible. Even when relocation is attempted, such as the case in Shishmaref, Alaska, which is affected by rapid barrier island migration and coastal erosion, the planning can last for more than 10 years and the relocation costs are projected to be at least about $180 million (Marino 2012 ). Erosion prevention structures are still in need even in those plan-to-move communities to help reduce damages to current civil infrastructures while planning and implementation take place. Therefore, there exists an urgency of identifying potential adaptations for northern high-latitude coastal erosion and change. One method of identifying potential adaptations is through a systematic review on the prevention of coastal erosion in key northern high-latitude areas.
Coastal stabilization projects have been one of the most challenging types of construction faced by contractors globally due to the complexities of various environmental forcing factors such as storm impacts, sediment transport, and deposition patterns. Site specific risks, which are sometimes difficult to be distinguished from other factors, can lead to catastrophic failures of the coastal protection structure in question. Errors in field investigations, design, construction, and maintenance can also reduce the effectiveness of the measures. In addition, extremes of climate and weather, which are changing from historical values (Ayyub 2018 ), introduce more uncertainties to coastal engineering practice at local levels. Such projects, when located at northern high latitudes, are further complicated by the presence of sea ice, changes in sea ice extent, the harsh frigid environment, permafrost thaw, and thermokarst. Across the highly scattered scientific publications and gray literature, opinions and evidence regarding the effectiveness of long- and short-term high-latitude coastal erosion control vary greatly. While some studies (e.g. Ogorodov 2003 , Carter and Smith 2011 , Bronen and Chapin 2013 ) have argued that shoreline protection exacerbates land erosion on adjacent land, others (e.g. Andrachuk and Smit 2012 ) show evidence that erosion prevention measures are effective in the long run. A systematic review of northern high-latitude coastal erosion controls is therefore necessary to understand which measures have worked and which did not.
This paper reviews the current erosion control practices being applied globally, with a focus on northern high-latitude communities (primarily in Alaska due to data availability), and explores potential measures that can effectively prevent or slow coastal erosion in northern high-latitude communities. Within this paper we (1) summarize the challenges in constructing erosion control measures in northern high-latitude regions and the solutions proposed in the literature, (2) synthesize and conduct a meta-analysis on case histories from scientific and gray literature publications both in permafrost and non-permafrost regions, (3) discuss the rationale, benefits, limitations, and costs of applying each prevention technique, (4) explore emerging techniques and technologies, and (5) identify the knowledge gaps in prevention of coastal erosion at northern high latitudes. The innovative techniques include those that have been tested in full-scale, small-scale research projects, laboratory settings, and numerical models. The costs of the current coastal erosion prevention measures are included to reflect the unit cost increase over the years in Alaska. The goal of this research is to assess past attempts at prevention and control of erosion along northern high-latitude coasts, so that this review may be used as a reference for decision-making for mitigating the impacts of erosion in northern high-latitude coastal villages in the future.
2. Data compilation of erosion control measures
The case histories and research studies of erosion control projects in this study are compiled from journal and conference articles and government documents. The databases that have been searched include the Northern Region Projects by the Alaska Department of Transportation and Public Facilities (ADOT&PF), documentation of Alaska Baseline Erosion Assessment by U.S. Army Corps of Engineers (USACE), the Denali Commission Project Database, and the documentation of Emergency Watershed Protection Program by Alaska Natural Resources Conservation Service (NRCS). According to USACE ( 2009d ), other agencies such as the National Oceanic and Atmospheric Administration (NOAA), U.S. Geological Survey (USGS), Federal Emergency Management Agency (FEMA), Bureau of Land Management, and U.S. Forest Service only occasionally assisted with erosion control projects in the Alaskan coastal communities. As a result, most of the protection measures in the Alaskan coastal communities that are discussed herein are extracted from the USACE database.
3. General challenges of construction at northern high latitudes
There are many challenges in the construction in the remote northern high-latitude coastal regions, including remoteness of construction sites, limited construction material, and extreme environmental conditions (see summary in supplementary table S1 (available online at stacks.iop.org/ERL/0000/0000/mmedia )). Challenges can be grouped into three main categories: geographic challenges, engineering challenges, and socio-economic challenges. Geographic challenges include site remoteness, extreme weather, highly variable site conditions, and short construction period. The engineering challenges include unavailability of equipment and instrumentation, unavailability of local construction materials, and limited database (e.g. documentation of environmental parameters and soil parameters, design and construction guidelines of erosion control measures that are specific to permafrost coastlines, and case studies of well-engineered northern high-latitude coastal structures). The socio-economic challenges consist of policy inadequacy, low labor retention, and vandalism. Solutions that are proposed in this paper and by those from the literature are synthesized accordingly to each challenge in supplementary table S1.
4. Coastal erosion processes at northern high latitudes
Erosion control structures should be selected and designed according to the types of coastal settings at a site in order to optimize the structure performance. It is therefore important to understand coastal erosion processes that are specific to northern high-latitude sites. Permafrost-affected sandy beaches at northern high-latitudes are dominated by erosion processes common in non-permafrost regions such as steepening of foredunes and transport of beach sediments on gently sloping shores (Farquharson et al 2018 ). In contrast, where ice-rich permafrost is present, thermal abrasion is the main coastal erosion process. Aré ( 1988 ) defined thermal abrasion as erosion of ice-rich permafrost coasts due to combined mechanical and thermal action of waves at the under-water bluff base. Thermal abrasion then leads to the development of a wave-cut niche, leaving a cornice overhanging and eventually collapsing under its self-weight (Aré 1988 , Hoque and Pollard 2009 , 2016 ). This feature, which is unique to ice-rich permafrost coasts, is known as the block failure. Processes that are also unique to coasts affected by permafrost are thermally based and include thermal denudation and thermal settling. Thermal denudation is the destruction of shore cliffs under the action of thermal energy of air and solar radiation; while thermal settling is the deepening of littoral zone of the sea due to the thermal action of sea water, whose temperature depends on the air temperature, solar radiation, and ocean currents. As erosion progresses and leads to the landward migration of the shoreline, deeper terrestrial permafrost that persists below the level of coastal erosion may then become sub-sea permafrost (Overduin et al 2014 ).
The duration of coastal processes also varies seasonally and annually across the northern high-latitude coasts due to variability in the duration of sea ice coverage. In northern high-latitude regions not affected by sea ice, the open sea duration is year-long, similar to that at middle and low latitudes. In regions affected by sea ice, erosion is limited to the ice-free months but the degree of erosion is generally affected by seawater temperature and salinity and other arctic features such as pressure ridges, coastal geomorphology, and sea ice (Overduin et al 2014 ). Pressure ridges, if grounded with their keels extending to the nearshore bottom, can shelter shores from wave action and protect adjacent shorelines from further sea ice pressure (Taylor 1978 ). Nearshore profiles can easily be destabilized if the grounded pressure ridges are eroded by increased wave action owing to the decline in sea ice extent (Taylor 1978 ). Sea ice in various forms (e.g. ice pileup, landfast ice, and drift ice) can extensively modify the coastal morphology. For examples, ice pileup can gouge up sediments onto the land and restore beaches (Kovacs 1984 ) but can also erode the lower shoreface (Radosavljevic et al 2016 ) and damage coastal protection structures. Whereas, sea ice and landfast ice help prevent thermal abrasion (Mahoney et al 2007 , 2014 , Günther et al 2015 ). Unfortunately, the current decline in landfast ice and sea ice has rendered the permafrost coasts more susceptible to erosion. Such complex interactions among these arctic coastal features need to be accounted in the design of coastal protection structures.
In general, northern high-latitude coasts can be categorized into lithified (i.e. rocky) and unlithified coasts, depending on the regional histories of glaciation. Unlithified coasts, which have high ground ice content and are usually composed of fine-grained soils, are more susceptible to erosion. It was reported in Overduin et al ( 2014 ) that the annual mean rate of erosion (0.4–1.1 m/year) is the highest along the Beaufort and East Siberian coasts, where unlithified ice-rich low-height bluffs are the common coastal type. As expected, Canadian Archipelago, Greenland, and Svalbard, where lithified coasts dominate, have the lowest annual mean rates of erosion (0.0 m/year) (Overduin et al 2014 ). While the mean rates at these locations are low, it is important to note that they are averaged over a great distance of coastline and may not represent the local erosion.
5. Past attempts to improve understanding of coastal dynamics and site reconnaissance for mitigating coastal erosion at northern high latitudes
Understanding of high-latitude coastal dynamics is critical to the design of effective erosion control measures. In the early 2000s, the Arctic Coastal Dynamics (ACD) program involving all circumarctic countries was established and has significantly contributed to the understanding of such dynamics through a series of workshop proceeding publications. Arctic coasts have been regionally classified and correlated with their rates of erosion; attempts have been made to identify arctic coast types most susceptible to erosion. Along the Alaskan Beaufort Sea coast, Jorgenson and Brown ( 2005 ) concludes that exposed bluffs have the highest mean annual erosion rates (2.4 m/year), whereas lagoons have the lowest rates (0.7 m/year). Mean annual rates of erosion is also higher for silty soils (3.2 m/year). The sediments are mostly sands and silts and the bluff heights are low (2–4 m) (Jorgenson and Brown 2005 ). Moving eastward to the Beaufort-Mackenzie region in Canada (Solomon 2005 ) and Barents and Kara Sea key sites in Russia (Vasiliev 2003 , Vasiliev et al 2005 ), locations exposed to winds and high water level were also observed to erode at the highest rates. Erosion rates are also higher for coasts with high ground-ice content (Vasiliev et al 2005 , Solomon 2003 ). However, erosion can be reduced if there are natural protections such as bars and flats (Solomon 2005 , Gibbs and Richmond 2017 ).
Many have attempted to understand coastal dynamics along sea-ice and permafrost-affected coasts so that erosion and its impacts can be mitigated. Attempted approaches include developing an index of coastal erosion hazards (Solomon and Gareau 2003 , Jordan 2003 ), utilizing satellite data for coastal erosion monitoring (Budkewitsch et al 2004 ), developing numerical models (Hoque and Pollardz 2004 ), seismic reconnaissance (Skvortsov and Drozdov 2003 ), and digital terrestrial photogrammetry (Wangensteen et al 2007 ) to predict bluff failure and erosion rates. Other than the environmental forcing factors, impacts of industrial exploitation on coastal erosion have also been investigated (Rivkin et al 2003 , Ogorodov 2003 , Sturtevant et al 2004 ). Significant efforts have been made to quantify the current and historical erosion rates across the pan-Arctic (e.g. Jones et al 2009a , 2009b , Arp et al 2010 , Solomon 2005 , Lantuit and Pollard 2008 , Vasiliev et al 2005 , Lantuit et al 2011 , Günther et al 2012 ) so that hotspots of erosion hazard can be identified. Challenges of site investigation (Carter and Smith 2011 , Smith and Carter 2011 ), data collection (Mason et al 2012 ), construction (Carter and Smith 2011 , Smith and Carter 2011 ), and management (Smith 2008 ) in coastal regions in the Arctic were also reported.
6. Structural and non-structural erosion control measures
Damages from coastal erosion can be minimized by two different approaches: structural and non-structural measures. This paper includes only structural measures, which are defined in the United Nations General Assembly ( 2016 ) as measures that 'reduce or avoid possible impacts of hazards and achieve hazard-resistance and resilience in structures or systems.' Non-structural erosion control measures that 'use knowledge, practice or agreement to reduce risks and impacts' (United Nations General Assembly 2016 ) are not discussed in this paper. Some examples of the non-structural measures are joining the National Flood Insurance Program (Smith 2008 ), implementing No Adverse Impact (Monday and Bell 2007 ), zoning, buyout acquisition, recovery or excavation of cultural sites, remediation of contaminated sites, relocation or elevation of at-risk structures, emergency warning system and signage, erosion control management, graduate education (Smith 2006 ), individual research efforts (Smith 2006 ), and community-based relocation (USACE 2018 , Shearer 2012 , Marino 2012 , Bronen and Chapin 2013 , Gorokhovich et al 2013 , Maldonado et al 2013 , Bronen 2015 ).
All structural erosion control measures presented in this article are classified into several categories as shown in figure 1 . Two major categories are (A) techniques that are currently employed on northern high latitude coasts and (B) techniques that are not yet tested on northern high latitude coasts. Sub-categories that follow Categories A and B are techniques that have been implemented (1) in full scale, (2) in small-scale research projects or physical models, (3) in laboratories or numerical models, and (4) in small scale non-engineered projects. In this study, a structure is considered failing when it has been destroyed or displaced by environmental impacts (e.g. wave impacts, surges, sea-ice impacts, and permafrost thaw) and the repair would cost more than replacement. Another failure type refers to the ineffective measures where erosion exceeds the expected or predicted rates of erosion. A successful case refers to a measure that is not damaged or only slightly damaged when the encountered storm events are less severe than or equally severe to the design events. A measure is also considered successful if it is able to slow down or prevent erosion within the period of its designed service life when compared to adjacent sites with no coastal protection.
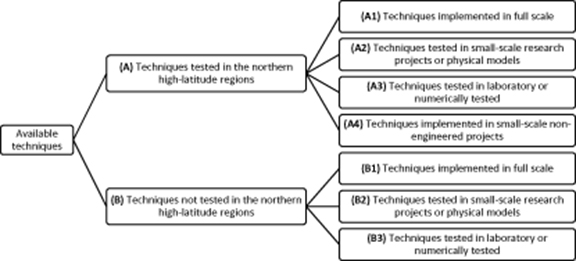
Figure 1. Categories of erosion control status used in this review.
Download figure:
7. Current erosion controls at northern high latitudes
Most case studies presented in this section are affected by coastal erosion while some are affected by fluvial erosion. Although these riverine communities are not affected by sea-ice and other processes specific to the coasts, the erosion control projects are affected by some similar factors unique to the northern high-latitude regions including remoteness, weather, and soil condition. So, the riverine experience may be helpful to the coastal communities and is therefore included in the discussion. The locations of northern high-latitude communities and facilities are presented in figure 2 with permafrost distributions as the base map. Figure 2 is adapted from the permafrost distribution maps by Brown et al ( 2002 ) and Jorgenson et al ( 2008 ).
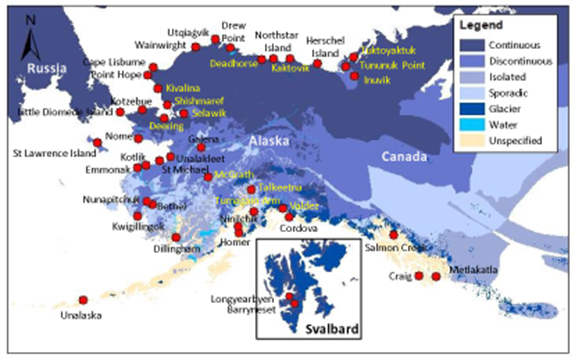
Figure 2. Locations of northern high-latitude communities and facilities. The locations are indicated as red markers on the map of permafrost distributions. The Alaska permafrost map is adapted from Jorgenson et al ( 2008 ), and the permafrost map outside of Alaska is adapted from Brown et al ( 2002 ). The sites in Svalbard are presented in the bottom frame. The legend indicates the permafrost distributions (i.e. continuous permafrost, discontinuous permafrost, isolated permafrost, and sporadic permafrost), glacier, water body, and unspecified regions.
Most of the measures that have been employed in Alaskan coastal villages and other infrastructure locations are hard structures such as revetments, bulkheads, seawalls, groins, and offshore berms. Figure 3 shows examples of these measures at northern high latitudes. The permafrost distributions (i.e. continuous, discontinuous, sporadic, and isolated permafrost) were identified for each site as indicated as superscripts in the column titled 'Location' in supplementary table S2. Thus far, revetments built with various types of materials have been the most common option in preventing coastal erosion at northern high latitudes as presented in supplementary table S2. The materials include rocks (figure 3 (a)), sandbags (figure 3 (b)), articulated concrete mats (figure 3 (c)), Core-loc™ units, timbers, and gabions. Among all materials, revetments built with rocks have been implemented and tested in the harsh Arctic environment and were observed to have fewer failure cases. Revetments built with sandbags have also been proven effective as temporary and semi-permanent measures. USACE ( 2009c ) has conducted physical models to further optimize the stability of revetments built with sandbags. In general, revetments usually require maintenance throughout their service life as they can be easily displaced and destroyed during storm events or by sea-ice floes. Despite this limitation, revetments are preferred over seawalls, bulkheads, and other offshore structures due to the low costs and easy construction, inspection, and decommissioning (USACE 2018 ).
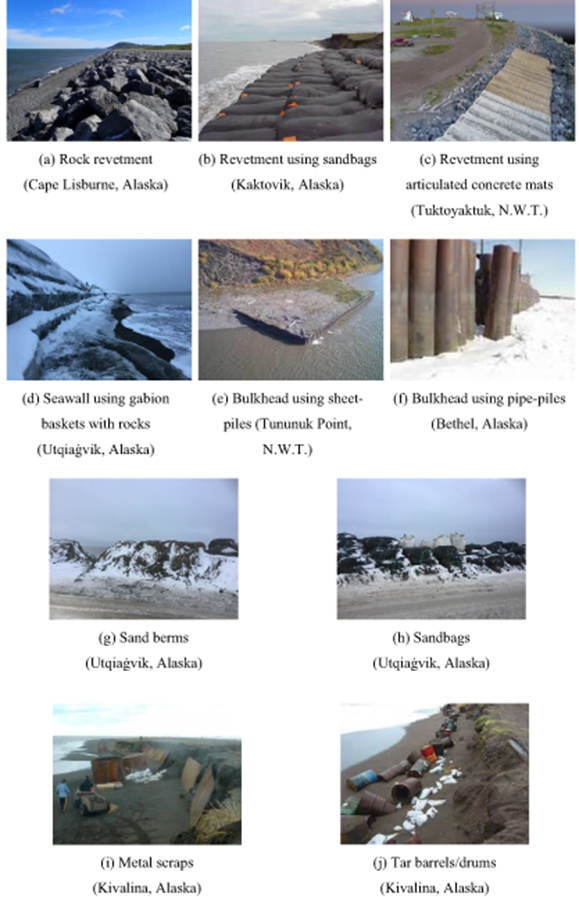
Figure 3. Various types of erosion control measures that have been employed at northern high latitudes. The photos were all taken at communities in the northern high-latitude regions. Image courtesies of U.S. Army Corps of Engineers, Alaska District (a), (b), (f), Dustin Whalen (c), Alaska Division of Homeland Security and Emergency Management (d), Worley Parsons (e), Millie Hawley (©2005) (i), and Janet Mitchell and Center for a Better Life (j). (g) to (j) are non-engineered expedient measures carried out during emergency.
Seawalls (figure 3 (d)) and bulkheads (figures 3 (e)–(f)) have also been constructed in northern high-latitude coastal communities. Similar to revetments, bulkhead and seawalls protect permafrost bluff faces from the development of niches, a critical stage in shoreline collapse (Hoque and Pollard 2009 , USACE 2018 ). For bulkheads, only one successful case using vertical sheet-piles is found in the literature review (USACE 2009a ). Bulkheads made of Longard tubes™ and pipe-piles were easily subjected to vandalism (Shah 1982 ) and excessive deflection (USACE 2007a , 2009d ), respectively. Whereas, one successful case using a seawall made of logs is found to be effective in mitigating fluvial erosion where the crib walls were constructed (USACE 2007h ). Seawalls made of gabions were not as effective due to potential sagging and settlement (USACE 2009b ); while seawalls made of sheet-piles were not cost-effective, for example, a sheet-pile wall in a project proposal was estimated to cost more than $31 million (in 2007 US dollars) for a 1 km long coastline (USACE 2007k ). The groin system is a less common option in northern high-latitude coastal communities with only one case study presented in supplementary table S2. Due to their complex offshore construction, groins are especially vulnerable to damage from sea-ice (USACE 2018 ). This paper is unable to identify a successful case study that used groin systems alone to mitigate erosion impacts. The erosion in Tuktoyaktuk in northwestern Canadian Arctic was reduced when the groin systems were installed together with bulkheads (Shah 1982 ). Similar to groin systems, offshore berms are not preferred at northern high latitudes due to costly and complex offshore construction. This option was once considered in the feasibility report in Kivalina but was eventually eliminated (USACE 2007k ). Overall, failures of seawalls, bulkheads, and groin systems are associated with displacement, deflection, settlement, vandalism, and material ruptures as presented in supplementary table S2.
In the event of an emergency, sacrificial structures have been constructed as expedient measures to minimize the erosion as shown in figures 3 (g)–(j). The materials used in sacrificial structures include sandbags, supersacks, ripraps, sand berms, and root-wads. Although the materials are the same as those used in the construction of hard structures, these sacrificial structures are ineffective when they are not properly designed and constructed. When in short supply, these materials are substituted using scrap metal and concrete and other waste materials. Such materials can potentially cause pollution. Based on the case histories presented in supplementary table S2, these measures are often ineffective in preventing or slowing erosion and require maintenance after each storm event (USACE 2007a , 2007d , 2007f , 2007g , 2007 j, 2008b , 2018 , Jaskólski et al 2018 ) and may sometimes exacerbate the adjacent coastline due to material mining (Carter and Smith 2011 ). Root-wads and willow planting are the only case histories that have been effective in preventing erosion, but the project is located in Cordova, which is a non-permafrost region of Alaska affected by riverbank erosion as shown in table S2 (USACE 2007a , Smith 2008 ).
Soft structures such as beach nourishment and dynamically stable beaches have also been implemented at northern high-latitudes. Beach nourishment requires continual sources of sand and is effective only when there are existing sources of sand adjacent to the sites. This solution is rarely used in northern high-latitude communities given the lack of local sand sources, transportation challenges and costs, the depletion of local construction materials, and the environmental issues brought by sand mining. As a result, only a few beach nourishment projects have been carried out along northern high-latitude coasts and these are presented in supplementary table S2. Beach nourishment is effective in Homer, Alaska (a non-permafrost region), but ineffective in Utqiaġvik, Alaska (a continuous permafrost region) where the storm events are more intense and sea ice can interfere with sand transport of the nourished beaches.
The other soft structure that has been implemented in northern high-latitude coastal communities is the dynamically stable beach. Specifications of the three dynamically stable beaches constructed in Alaska were presented by Smith and Carter ( 2011 ) and are summarized in supplementary table S2. Coarser sediments such as rocks, concrete armor units, and cobbles were used. The main difference between a dynamically stable beach and a nourished beach is that the former is designed according to the wave direction and is designed to be shaped by future storm events to reach equilibrium over time. Only minimal maintenance is needed for dynamically stable beaches, depending on the intensity of the storm events, whereas another beach-filling is needed for the nourished beach to continue its function after the first storm event. According to Smith and Carter ( 2011 ), all three dynamically stable beaches (i.e. one in Unalakleet, a village located on a spit near a river mouth in the discontinuous permafrost region of Alaska, and two in Unalaska and Homer in the non-permafrost affected region) have performed well and do not require high maintenance after storm events.
8. Meta-analysis of current measures with site characteristics
8.1. coastal landforms.
Alaskan coastal communities mostly reside at landforms such as bluffs with narrow beaches, spits, islands, barrier islands, and river mouths. Among the various landforms, spits are the most frequently resided (5 out of 13 locations collected in this study). In figure 4 , the frequencies of northern coastal communities at various coastal landforms are plotted in light gray; the frequencies of erosion control case studies (total number n = 32) collected in this study were plotted in dark gray. The frequency of the case studies indicate that more measures had been repeatedly constructed in barrier islands and spits. In general, the soil materials in villages range from silts, fine sands, sands, to cobbles. Little Diomede Island, which is an exception, is rich in rocks and boulders.
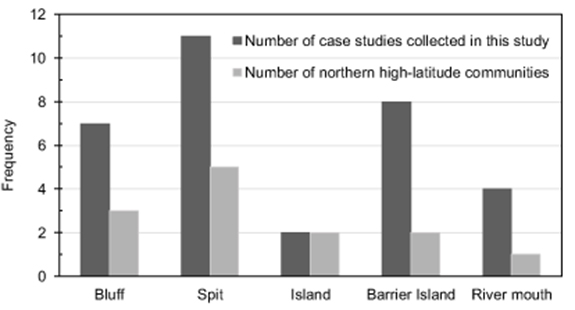
Figure 4. Frequencies of the northern high-latitude coastal communities and case studies for various coastal landforms. The y -axis represents the frequency counts of the numbers of villages or the numbers of case studies collected in this study. The raw data used to compute the bar chart are obtained from the literature, mostly from Alaska Baseline Erosion Assessment Reports (UMIAQ and BDS 2015 , USACE 2007b , 2007d , 2007k , 2007l , 2009a , 2007f , 2007g , Jaskólski et al 2018 ).
8.2. Rates of erosion
Figures 5 (a)–(b) shows boxplots of the mean annual erosion rates and erosion per single storm event reported at sites where the erosion controls were implemented. Numeric data used to generate the plots are included in supplementary table S3. As illustrated in figure 5 (a), revetments and bulkheads have been employed for sites with a wide range of mean annual erosion rates (0.3–2.4 m/year). As expected, groin systems, which are an offshore structure, have been employed at sites with high mean annual erosion rates (2.3 m/year); whereas, beach nourishment, dynamically stable beaches, and other non-engineered measures have been employed at sites with low mean annual erosion rates (0.3–0.9 m/year). However, seawalls were employed at sites with low erosion rates (0.3–0.6 m/year). It could be possible that seawalls were constructed in these sites to slow erosion after extreme episodic erosion (figure 5 (b)). Similarly, for revetments and bulkheads, the erosion reported per single storm event also ranged widely from 3.0 m to 22.9 m. The episodic events of erosion were at the lower ends for beach nourishment, dynamically stable beaches, and other non-engineered measures (6.0–10.7 m) with an outlier of 22.9 m for non-engineered measures. Seawalls, groins, and berms had been constructed at sites with high episodic erosion (17.0–22.9 m).
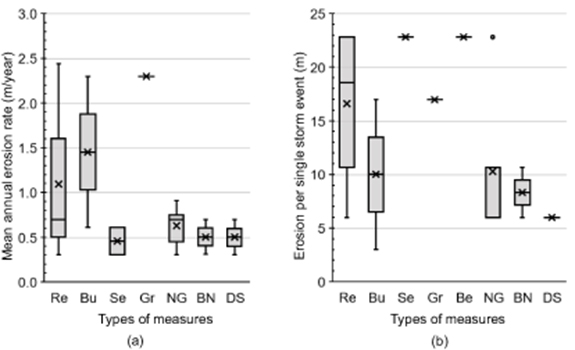
Figure 5. Statistical distributions of erosion rates at sites where erosion control measures were employed. (a) Mean annual erosion rates in m/year. (b) Amount of land eroded per a single storm event during the extreme cases. For the types of measures, Re = revetments, Bu = bulkheads, Se = seawalls, Gr = groins, Be = berms (no mean annual erosion rate was reported for berms), NG = non-engineered measures, BN = beach nourishment, and DS = dynamically stable beaches. Mean values are indicated as cross markers, while outliers are empty circles. Medians are indicated by the horizontal lines in the boxes. The raw data used to compute the boxplots are obtained from the literature, mostly from Alaska Baseline Erosion Assessment Reports (UMIAQ and BDS 2015 , USACE 2007b , 2007d , 2007k , 2007l , 2009a , 2007f , 2007g , Jaskólski et al 2018 ).
8.3. Proportions of various types of measures
Figure 6 (a) shows the proportion of the types of erosion control measures employed in the northern high-latitude coastal and riverine communities. Based on a total of 53 cases, revetments were most frequently employed (41%) and non-engineered measures were the second (23%). The measures that came after non-engineered measures are seawalls (11%), bulkheads (9%), dynamically stable beaches (8%), beach nourishment (4%), groins (2%), and berms (2%). Among the 53 cases at northern high latitudes, 38 of them were employed within permafrost regions (including continuous, discontinuous, sporadic, and isolated permafrost). For the rest of the cases ( n = 15), although they are influenced by northern high-latitude climate, they are located within non-permafrost regions. For the cases in permafrost regions, the percentage of revetments increases to 44% whereas the percentage of non-engineered measures reduces to 16%. Similarly, figure 6 (b) shows the proportions of the types of materials. Among all the cases collected in northern high-latitude regions, riprap is the most frequently used material, accounting for 40% of the materials. Sand or sandbags and sheet piles are both the second (11% for each). For cases in permafrost regions, the percentage of riprap reduces to 34%, but both percentages of sand or sandbags and sheet piles increase to 13% and 16%, respectively. The percentage differences between counts in northern high-latitude regions and those in permafrost-affected northern high-latitude regions are indicated on the pie charts in brackets. The percentages of the measures (in table 1 , the column 'Grouped percentages') computed in this study are compared to those (in table 1 ; the last column) reported in a survey 'Alaska Community Erosion Survey' conducted by USACE ( 2009f ). In this survey (USACE 2009f ), the Alaskan coastal communities were asked to indicate the types of materials that have been used in their communities to prevent coastal erosion. The results reported in this paper, which were computed based on case studies reported in the literature, were comparable to the survey with riprap (including rocks and cobbles) being the most frequently used measures and geo-tubes being the least popular option.
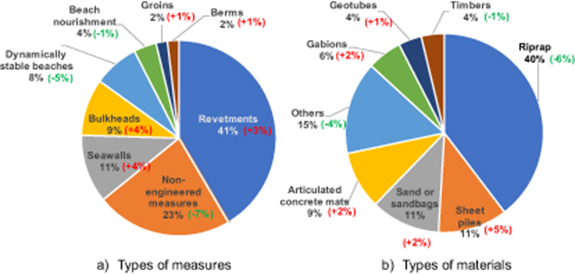
Figure 6. Proportion of types of (a) erosion control measures and (b) materials employed in northern high-latitude communities. Values not in brackets represent proportions for all case studies at northern high latitudes; the values in brackets are the comparisons with those in permafrost areas. Values in red indicate higher percentages for the cases in permafrost regions compared to all northern high-latitude cases, and vice versa for values in green.
Table 1. Percentages of various types of measures discussed in this study and percentages of measures covered by the 2009 USACE survey data.
8.4. Effectiveness of current measures reported in community survey
In the same survey by USACE ( 2009f ), the communities were asked about the effectiveness of the erosion control measures currently employed in their communities; 84% of the community respondents ( n = 44) indicated that the measures had been effective. The communities were further asked about whether there had been a failure in the past in their communities; 100% of the respondents ( n = 23) indicated that there had been a failure. In general, the communities reported that the current measures cannot fully prevent erosion, are not adequate for large scale protection, and have been heavily damaged due to lack of repair and maintenance. It is likely that these past measures were designed without adequate site investigations and without the considerations of the changing climate. As a result, these measures were only able to prevent mild constant erosion but not extreme erosion in an episodic manner and may fail in future storm events.
9. Costs of erosion control measures at northern high latitudes
The cost of erosion controls in Alaska has been escalating over time even for the expedient measures that were built during the planning of community relocations such as those in Kivalina and Shishmaref (USACE 2009d ). Supplementary table S4 shows the type of protection measure for each case history and the corresponding length of the structure, cost, and year of construction. The map of permafrost distributions developed by Brown et al ( 2002 ) and Jorgenson et al ( 2008 ) were used to identify permafrost distributions (i.e. continuous, discontinuous, sporadic, and isolated permafrost) for each site as indicated as superscripts in the column titled 'Communities in Alaska' in supplementary table S4. The cost per meter of erosion control measures that have been implemented or recommended in the feasibility studies are plotted in figure 7 over 40 years from 1979 to 2018. Inflation is accounted by converting the costs to 2019 US dollars using the Consumer Price Index Inflation Calculator developed by the U.S. Bureau of Labor Statistics ( 2019 ). It is important to note that the impacts and concerns on coastal communities and facilities predate the 40-year time interval, dating back to the well-known 1963 storm event in Utqiaġvik, Alaska; this storm event is widely used as a historical reference in the North American literature. However, this 40-year time interval was selected mainly because of the availability of reports and documents specific to coastal protection structures. The early review paper by Aré ( 1988 ) might have inspired more efforts (and therefore more documentation) to be made in the field of coastal erosion protection since then.
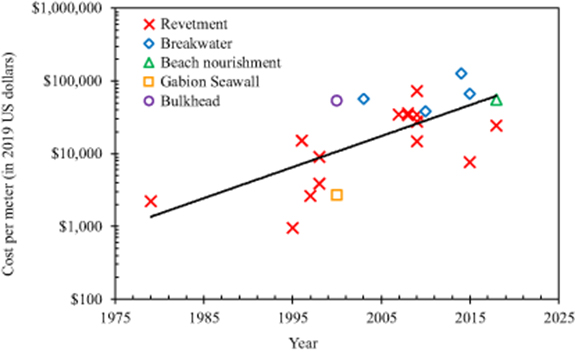
Figure 7. Cost per meter of coastal erosion controls from year 1979 to 2018 along Alaskan coasts. Inflation is accounted by converting the costs to 2019 US dollars using the consumer price index inflation calculator developed by the U.S. Bureau of Labor Statistics ( 2019 ).
As shown in figure 7 , the unit cost has increased (approximately 10% increase per year) in the past four decades. The costs of breakwaters (figure 7 , blue diamond-shaped markers) and bulkheads (figure 7 , purple circle marker) are above the average cost. The cost of gabion seawall construction is below average and beach nourishment falls on the average cost. Although the estimated cost of the beach nourishment seems to fall within the median range as shown in figure 7 , its cost is considerably high when compared to the average cost in mid- to low-latitude regions. For an 8 km shoreline in Utqiaġvik, AK (USACE 2018 ), for example, the initial cost of the beach nourishment was estimated at $297 431 000 (in 2018 dollars). This results in a cost of approximately $37 000 per meter for initial construction. However, in mid- to low-latitude regions, the initial construction cost for beach nourishment ranges from only $6600–$16 000 per meter of coastline (SAGE 2015 ). This infers that the initial cost to practice beach nourishment in a northern high-latitude region is at least 2 times the cost needed for a mid- to low-latitude region. Similarly, in mid- to low-latitude regions, the initial construction cost for bulkheads ranges from $6600–$33 000 per meter (SAGE 2015 ). However, the bulkhead in Dillingham, Alaska, which is a northern high-latitude village affected by fluvial erosion, costs about $53 000 per meter in 2019 US dollars (USACE 2009d ). This is equivalent to a cost that is approximately 1.6 to 8 times higher than the cost needed for a similar application at middle to low latitudes.
9.1. Meta-analysis of cost of erosion control measures
A statistical analysis was further performed on the case studies with reported construction cost (figure 8 ). As expected, breakwater, which is an offshore structure, has higher median cost than the other measures (figure 8 (a)). Such trend is much clearer when comparing breakwater to revetment but not the other measures since only one data point is available for bulkhead, seawall, and beach nourishment. The low unit cost of revetments may be one of the reasons that lead to the popularity of revetments (as illustrated by the high frequency of revetment in figure 8 (b)) in controlling coastal erosion in Alaska.
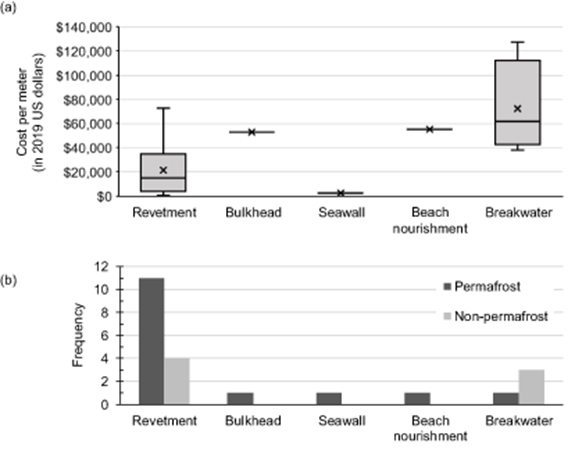
Figure 8. Statistical distributions of (a) unit cost in 2019 US dollars and (b) how frequently various types of coastal erosion controls are implemented. For the boxplots, mean values are indicated as cross markers; medians are indicated by the horizontal lines in the boxes. The vertical axis of chart (b) refers to the number of case studies.
Although breakwater, which is mostly constructed in non-permafrost regions, has a higher median cost, the cost (both unit and total costs) of erosion control measures in permafrost regions is higher (in figures 9 (a) and (b)). This is likely because a revetment costs more if constructed in a permafrost region, driving up the cost of overall measures. In addition, it is noted in figure 9 that the difference in medians between permafrost and non-permafrost is higher for the unit cost but not as much for the total cost. This is because the length of coastline protected by the measures in non-permafrost regions is generally longer.
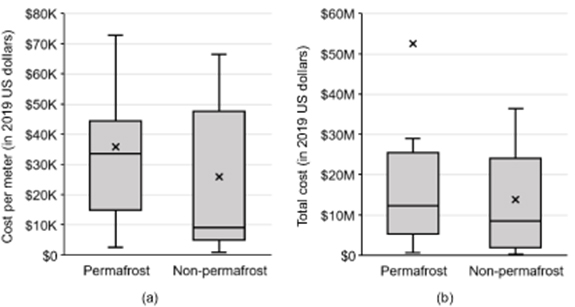
Figure 9. Comparison of costs (in 2019 US dollars) of coastal erosion control in permafrost ( n = 15) and non-permafrost ( n = 7) regions. (a) Unit cost. (b) Total cost. Mean values are indicated as cross markers. Note: the mean total cost for measures in permafrost regions is skewed by an outlier (i.e. a beach nourishment project that was proposed in Utqiaġvik in 2018 and was projected to cost about $439 M in 2019 US dollars), which is not presented in the chart, so that the boxplots can be better illustrated.
10. Potential erosion control measures or techniques
The potential prevention measures or techniques that have been used in mid- to low-latitude regions or assessed for their potential application in Arctic systems are synthesized and categorized into four different applications: geosynthetics, static bay beach concept, refrigerating techniques, and biogeochemical application. Geosynthetics have been implemented in full-scale applications in various forms to control coastal erosion in mid- to low-latitude regions as shown in supplementary table S5. They were used as offshore structures (e.g. breakwaters) and onshore structures (e.g. sand-bagged seawall, sand-bagged revetments, and wrap-around revetments). Martinelli et al ( 2011 ) observed that high-density polyethylene (HDPE) sandbags when constructed as submerged barriers resisted a strong storm surge in Emilia-Romagna, Italy in December 1996 and stabilized a natural sandy beach profile. When constructed as a seawall, the sandbags resisted a significant wave height of 5 m (Corbella and Stretch 2012 ). Another type of geosynthetic application, revetments constructed using Geotube® units, prevented erosion at a localized segment of coastal bluff (Nickels and Heerten 1996 , Yasuhara et al 2012 ). Geotube® units can be a potential protection measure during emergencies given its short construction duration of less than one hour per Geotube® (Shin and Oh 2007 ). Another emerging geosynthetic application is geotextile wrap-around revetments (GWR). The GWR structure in Sylt Island in Germany was effective in resisting wave action during intense storm events in 1993 and 1994 and prevented coastal erosion by more than 10 m when compared to adjacent coastlines (Nickels and Heerten 1996 , Yasuhara and Recio-Molina 2007 , Yasuhara et al 2012 ). Although the sand-covered structure was exposed after the storm events, it was not damaged. Small-scale geosynthetic applications incorporating geotextile bags with conventional methods (e.g. breakwater) have been implemented in some northern high-latitude sites. The geotextile sandbags were designed and installed in Longyearbyen and Barryneset in Svalbard in 2005 for an experiment to develop geosynthetics products for cold climates (Caline 2010 ). The USACE ( 2009c ) started to consider using geosynthetic applications along the Alaskan coasts given the successful case studies across low to high latitudes.
The static bay beach concept (SBBC), as synthesized in supplementary table S6, is similar to the dynamically stable beach presented in supplementary table S2. This application mimics the shapes of natural bays and beaches. It prevents erosion by allowing a shoreline to reach static equilibrium through incorporations of natural or artificial physiographic features (preferably a headland). Several case studies in northern high-latitude regions have demonstrated the effectiveness of the static bay beach concept. While the principle employed is the same at both the permafrost and non-permafrost sites, relatively coarse fill materials were selected and used for the permafrost sites in order to dissipate greater wave energy. The benefits of the static bay beach are its low costs and its satisfactory performances in the long term when compared to other hard measures (Hsu et al 2010 , Carter and Smith 2011 ).
Refrigerating systems such as thermosyphons have traditionally been utilized at permafrost sites to improve the stability of roadbeds and embankments (Regehr et al 2012 ). There are three different types of thermosyphons: active, passive, and hybrid. An active thermosyphon uses a heat pump to transfer heat (Wagner 2014 ). A passive thermosyphon uses working fluid to transfer heat from its evaporator (in the ground) to its condenser and radiator (above the ground) when air temperature is lower than the ground temperature (Gudmestad et al 2007 ); this heat transfer process does not utilize external power and ceases during the summer when the air temperature is higher than the ground temperature. A hybrid thermosyphon integrates the functions of both active and passive thermosyphons and operates with natural convection during winter months (when air temperatures are sufficiently low) and with a heat pump during summer months (when air temperatures are above 0 °C). According to Dupeyrat et al ( 2011 ), an increase in ground temperature can cause the frozen ground to thaw. Such phase change of ice within the permafrost results in excess water content, which in turn reduces the cohesion and shear strength of permafrost. So, the erosion resistance of thawed soil decreases; as a result, the rate of erosion increases. In brief, erosion can be potentially prevented or reduced if ice-bonded sediments can be kept below its freezing point. To date, this technique has only been applied in small scale to improve the stability of critical infrastructure such as the communication towers in Kwigillingok, Alaska, the power plants in Utqiaġvik, Alaska, the hangar facility in Deadhorse, Alaska, and the college in Inuvik, Canada (Wagner 2014 ). Recently, a thermosyphon system with 3 meter spacings was also proposed to mitigate river erosion in Kotlik in the sporadic permafrost zone of Alaska, but the proposal was eventually eliminated due to the high cost (Roberts et al 2019 ). Although conventional thermosyphons (typically installed with 3 m spacings) cost about $1 M per km, they require minimal maintenance throughout their service life. Zottola ( 2016 ) proposed to use two-phase passive thermosyphons to alleviate coastal erosion through freezing near-thawing soils. The numerical models with soil and climate data from Drew Point and Utqiaġvik, Alaska as input parameters showed that thermosyphons are capable of slowing permafrost coastal erosion (Zottola 2016 ) but further refinement of the design is needed to optimize its cost. Detailed information for the case studies of thermosyphons are summarized in supplementary table S7.
The microbial application is an emerging technique that has been applied to control internal and surface erosion and its overall effectiveness has been rated highly (Dejong et al 2013 ). Many research studies have focused on developing the technique to be ready for real-world applications, and some studies have begun to investigate the performance of microbial application at coastal bluffs and sand dunes to mitigate coastal erosion (Phillips et al 2013 , Imran et al 2019 , Shahin et al 2020 ). Detailed information of the two case studies that may be applicable to the northern high-latitude sites are presented in supplementary table S8. One of them is a bench-scale project, investigating the performance of sporosarcina pasteurii in mitigating erosion of sandy soils of foreshore slopes and sand dunes (Salifu et al 2016 , Shanahan and Montoya 2016 , Shanahan 2016 ). Sporosarcina pasteurii is a bacterium with the ability to precipitate calcite and cement sand particles given a calcium source and urea, through the process of microbiologically induced calcite precipitation (MICP) or biological cementation. The treated soils showed above-moderate unconfined compressive strength and improved the performance of sand dunes under wave-simulation. The wave-action-induced erosion was significantly reduced when the sand was treated with a microbial solution (Salifu et al 2016 ). The other study was conducted using native microbes to cement sand to develop artificial beach-rocks that are durable enough to replace concrete structures in erosion control projects (Khan et al 2015 , 2016 ); coral sand and ureolytic bacteria from beach-rocks in Nago, Japan were used in the study. An average unconfined compressive strength of 12 MPa was achieved for the artificial beach rocks after 14 d of curing. This technique can potentially resolve the shortage of local quarries in remote northern high-latitude villages and control the escalating cost of rocks and ripraps.
11. Research gaps and challenges
To date, the potential erosion control measures and techniques described in the previous section have not been fully tested in the northern high-latitude regions. Some of them have not been evaluated even under the laboratory-simulated northern high-latitude conditions. Here we assess their strengths and weaknesses within the northern high-latitude environment and identify research gaps that need to be filled before field implementation.
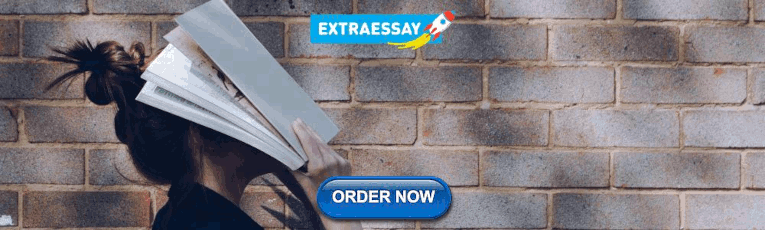
11.1. Geosynthetics
Among the potential erosion control measures and techniques that we researched, geosynthetics currently have the greatest potential to be applied along the northern high-latitude coasts given their successful field applications in the past. Erosion has been controlled using geosynthetics from sparsely-located individual geosynthetic sandbags to well-designed seawalls constructed using geosynthetic materials. However, the main challenge of using geosynthetics is the prolonged UV exposure by 24 h daylight a day in the summer. Developing UV resistant and non-degradable geosynthetics is needed for northern high-latitude applications. Geosynthetic materials, which can be easily decommissioned, may be an effective option for temporary erosion control so that the threats to environment can be reduced (Sinitsyn and Recker 2019 ). However, if not properly monitored, the degraded geosynthetic materials can pollute the environment, posing threats to arctic wildlife. The degradation of geosynthetic materials in harsh and frigid northern high-latitude environments (e.g. impacts of ice-floes and prolonged exposure to subzero temperatures) can further complicate the problem by allowing brittle ruptures of sandbags to occur, resulting in sand leaking and excessive sand movement within the sandbags. Settlement and collapse of a seawall made of geosynthetic bags can occur if sand move excessively within those bags (Corbella and Stretch 2012 ). As a result, materials that remain flexible and non-brittle at low temperatures need to be identified; Caline ( 2010 ) therefore suggested that highly angular aggregates and those larger than 10 cm should be removed to avoid brittle puncture. Damage to geotextile sandbags by low temperatures or coastal ice can also be reduced by using thicker geotextile fabrics, but the seams on thick fabrics can be easily unpicked (Caline 2010 ). Therefore, the sewing machine and sewing threads need to be selected accordingly for the geosynthetics materials. In addition, the effects of sand movement on the overall stability of the geosynthetic structures need to be evaluated. The optimal sand-filled volume also needs to be determined for different combinations of locally-available filling materials and geosynthetics materials.
11.2. Static bay beach concept
One of the challenges of static bay beach concepts is to develop a process-based shape equation that can accurately simulate a northern high-latitude coastline with various coastal features. If simulated coastline profiles are not consistent with the real profiles, northern high-latitude beaches at static equilibrium will be difficult to maintain and final maintenance costs will vary substantially from their respective initial estimations. The current shape equation (i.e. Gainza et al 2018 ) is capable of modeling complex bathymetry incorporating influences of near shore islands, rock outcrops, and rocky platforms. For a shape equation to be effectively applied to a northern high-latitude coastline, effects of headlands, bluffs, barrier islands, spits, and ice ridges on the nearshore need to be considered in the equation. Another challenge is to identify the control points when applying a shape equation (Lausman et al 2010 ) as the final prediction of a static equilibrium shoreline highly depends on the initial selection. As those coastal features degrade due to thawing of permafrost or melting of ice, so do the controls points. The SBBC application also becomes more challenging when environmental forcing factors (e.g. sea ice, wave fetch, storm patterns) are changing due to climate change processes. Effects of these changes need to be reconsidered to predict the long-term equilibrium coasts at northern high latitudes.
11.3. Refrigerating system
The challenges of implementing thermosyphons are mostly associated with their up-scale field application in terms of high transportation costs and complex installation as compared with those of the traditional hard structures. Besides, given the high initial implementation costs of thermosyphons, they must be appropriately selected so that their capacities are flexible enough to account for the future climate and permafrost warming conditions. During summer months when air temperature is higher than the ground temperature, the heat pump of an active or a hybrid thermosyphon system needs to be activated to continue the heat transfer process. Heat pump operation may consume more energy if the mean annual air and ground temperatures continue to rise in a warming climate. The possibility of utilizing green energy such as the solar energy can be explored to lower the fuel consumption.
11.4. Microbial application
One of the concerns of applying MICP to the soils in northern high-latitude regions is the efficacy of the precipitate formation under cold temperatures. As reported in Cheng et al ( 2016 ), calcite can still form on grain surfaces at a temperature as low as 4 °C using Bacillus pasteurii although the amount of calcite formed per unit weight of sand at the temperature of 4 °C is 25% lower than that at 25 °C and the unconfined compressive strength is 56% lower. Performances of the calcite precipitation at 4 °C have also been assessed by Jiang et al ( 2016 ) using Bacillus megaterium and purified urease enzyme. B. megaterium was selected over Sporosarcina pasteurii as the former is more versatile and can grow at low temperatures (Jiang et al 2016 ). Efficacy of the calcite precipitation at temperatures lower than 4 °C needs to be evaluated in future studies to minimize the thermal disturbances to both the active layer and permafrost and to maintain the desired urealytic rates. Gomez et al ( 2015 ) developed a stiff crust, which has a high resistance to erosion, in the field using Sporosarcina pasteurii . This technique can be tried and tested at a bluff face to form a crust, which can potentially prevent niche development at the toe and insulate the permafrost beneath. However, the challenges include calcite degradation under freeze-thaw effects and wave impacts.
12. Choosing the optimal measures or techniques
The potential erosion controls are summarized in table 2 with their corresponding rationales, coastal types, tidal environments, and possible integration with conventional measures. The majority of the potential erosion controls are applicable to sandy coasts and are effective in a low energy setting. In the past Arctic coastlines (such as the North Slope of Alaska) were generally regarded as a low wave-energy environment where waves were damped by perennial sea ice cover (Owens et al 1980 ) these measures could be applicable to such shores. However, given that sea ice is now declining and high-latitude coastlines may transition into a more dynamic coastal system, these potential erosion controls may soon be inapplicable and need further development. Other than conventional solutions, the geosynthetic application could also be a good option for a high energy setting due to its several successful case histories in withstanding strong wave impacts and surges. Thermosyphon systems can also be used to dissipate high wave energy if integrated with sheet-pile walls or any equivalent measures. All of the potential erosion control measures and techniques discussed herein can be applied on the beach, except for the refrigerating systems, which are intended to be located on ice-rich permafrost bluff tops to keep them from thawing. The selection of erosion control measures is site-specific and is constrained by many factors such as cost, construction material availability, and contractors' skills and experience. At coastal sites where rocks are readily available, it may be more cost effective to continue to employ rock revetments to control erosion.
Table 2. Rationales of the potential erosion controls and the applicable soil types, coast types, and tidal environments.
Other than effectiveness, many factors (e.g. total costs, construction material availability, and sustainability) should also be considered in selecting the optimal coastal erosion control measures for a site. The life cycle cost analysis (LCCA), which is a quantitative approach that selects optimal measures based on their total costs over the life cycle, has been the primary framework used by USACE and construction companies. The total cost in LCCA includes the initial construction costs, annual maintenance and repair costs, operating costs, and inspection costs. However, analyses based on merely the total costs may not be adequate and the environmental impacts should also be considered. Recently, the life cycle assessment (LCA), which assesses environmental performance and impacts of a measure over its life cycle, including raw material extraction, manufacturing, use, disposal and recycling, are of rising interest in civil engineering (Singh et al 2011 , Dong and Frangopol 2016 , Raymond et al 2019 ). A combined assessment that incorporates both environmental and socio-economic impacts can be helpful for choosing the optimal coastal erosion control measures and should be transferred to coastal and geotechnical engineering in northern high-latitude regions.
13. Conclusions
This study investigates the conventional techniques that are currently used for northern high latitudes and emerging erosion control techniques applied globally that can potentially be adapted to prevent or limit coastal erosion in northern high-latitude regions. Challenges of implementing erosion prevention measures in northern high-latitude regions are summarized into three categories: geographical, engineering, and socioeconomical categories; the corresponding solutions, which are proposed in the literature, are also systematically documented in this review.
Meta-analysis is conducted on the case studies collected from scientific and gray literature publications. Our analysis shows that revetments built with rocks have the least reported failures among the current erosion controls and are the most common (41% of all measures) and cheapest measures applied in northern high-latitude settings, when compared to other less successful hard measures such as seawalls, bulkheads, and groin systems. Riprap is the most frequently used materials, accounting for 40% of the measures. No successful case history has been reported for the non-engineered expedient measures that are constructed in northern high-latitude regions in the event of an emergency. The effectiveness of beach nourishment as a soft structure in permafrost regions is inconclusive. However, dynamically stable beaches, which are also a soft structure, are effective in preventing erosion; observations show that they experience only minor damages after single storm events. Based on the collected case studies, we found that spits are landforms most frequently resided by the Alaskan coastal communities and more measures had been constructed on spits. Revetments have been implemented at sites with a wide range of mean annual erosion rates and episodic erosion due to the low costs and easy construction, inspection, and decommissioning.
By analyzing the cost of current erosion control measures in Alaska, in regions with and without permafrost, we show that the unit cost of erosion control structures has been escalating over the past 40 years (approximately 10% increase in cost per year). We also found that both the median unit cost and median total cost are higher for measures implemented in permafrost regions than those in non-permafrost regions; both permafrost and non-permafrost case studies in this cost analysis are located within the northern high-latitude regions.
The potential erosion control measures and promising techniques synthesized in this study include geosynthetics, the static bay beach concept, insulation systems, and microbial applications. The potential of these emerging measures and techniques for full-scale application on northern high-latitude coasts are discussed after reviewing the available research studies on their efficacy and performances under the high-latitude conditions, especially under the existence of sea ice and permafrost. Integrations of these potential measures and techniques with conventional measures are recommended and possible combinations are presented. We also propose to use a combined assessment (i.e. life cycle cost analysis and life cycle assessment) that incorporates both environmental and socio-economic impacts for optimal measure selections. This study shows that a wide knowledge gap still exists in the field application of the new measures and techniques in northern high-latitude regions even though some of them have already been widely implemented in mid- to low-latitude regions. Through the synthesis of the research gaps and challenges, future research can be directed towards upscaling of the emerging erosion control measures and techniques in northern high-latitude coastal regions.
Acknowledgments
This work was supported by the National Science Foundation under Grant Nos. OPP-1745369, OISE-1927137 and OISE-1927553. Any recommendations or conclusions are those of the authors and do not necessarily reflect the views of the US Government. The mention of trade names or commercial products does not in any way constitute an endorsement or recommendation for use. The authors would like to thank the six anonymous referees for their significant comments and constructive suggestions.
Data availability statement
The data that support the findings of this study are available from the corresponding author upon reasonable request.
Supplementary Data (361 KB, PDF)
UH Hilo Stories
‘A‘ohe pau ka ‘ike i ka hālau ho‘okahi │ One learns from many sources │ A publication from the Office of the Chancellor, University of Hawai‘i at Hilo
- UH Hilo on Facebook
- UH Hilo on Instagram
- UH Hilo on X (Twitter)
- UH Hilo on YouTube
Case study on UH Hilo research of coastal erosion published in “U.S. Climate Resilience Toolkit”
The innovative research combines historic aerial photos, current drone imagery, and topographic surveys to discover coastal changes around Hawai‘i Island. The results of the study are informing policymakers who are crafting a new setback policy for coastal development.

By Susan Enright .
The results of a collaborative research project led by a graduate student at the University of Hawai‘i at Hilo have been published as a case study in the U.S. Climate Resilience Toolkit , a website where the public can find information and tools to understand and address climate risks. The website offers information from all across the United States federal government in one easy-to-use location. The case study also has been published regionally on the Pacific Islands Regional Climate Assessment (PIRCA) website .
The UH Hilo study examines shoreline migration—the rate that changes occur, gradually or suddenly—on Hawai‘i Island and is being used by county planners and policymakers to develop a more comprehensive and effective coastal development plan.

The published case study, “Reality Check: Collaborative Research Contributes to Real-Life Policy Decisions,” is co-authored by Scott Laursen , program specialist at the UH Pacific Islands Climate Adaptation Science Center . The center was established by the U.S. Department of the Interior in 2011 as a consortium hosted by UH Mānoa, UH Hilo, and the University of Guam.
The case study is co-authored by Bethany Morrison , a planner on land use with the County of Hawai‘i who collaborated on the research.

“We do not have adequate knowledge of Hawai‘i Island’s shoreline to be able to assess and adapt to the vulnerabilities from sea level rise and related hazards,” Morrison explains. “The goals of this project will help us to address these challenges. More specifically, Hawai‘i County will have a first phase of shoreline change rates and sea-level rise projections for three different types of shorelines.”
Laursen says that local managers such as Morrison, who work with regional land and seascapes, are also working within the social norms and values of the communities that utilize those ecosystems.
“Directly involving local professional networks within every stage of the scientific method roots research products within the place-based experiences of these natural and cultural resource managers,” Laursen explains in an email. “[This] increases the probability that these products will be utilized. Resource managers like Bethany can be thought of as ‘custodians of context’ throughout the research process, ensuring the immediate utility of research output.”
The innovative research in this particular project combines historic aerial photos, current drone imagery, and topographic surveys to discover coastal changes around Hawai’i Island. The results of the study are informing policymakers who are crafting a new setback policy for coastal development.
UH Hilo responds to an urgent request from the county
In 2016, responding to an urgent need to reevaluate set back policy in county code, planning experts in the County of Hawai‘i Office of Planning initiated a collaborative two-year research project funded by UH Hilo’s Manager Climate Corps, a program of the Pacific Islands Climate Adaptation Science Center. A research team was assembled, made up of Morrison and researchers from UH Hilo, to examine and quantify historic and contemporary rates of change along different types of shorelines on Hawai‘i Island, and then to model observed changes into the future through sea level rise impacts.
On the research team was UH Hilo graduate student Rose Hart —who had completed an undergraduate internship involving research on shoreline setback policies—who was the driving force behind the project. Also on the team was Ryan Perroy , associate professor of geography and director of UH Hilo’s Spatial Data Analysis and Visualization Labs . Perroy is an expert and award-winning researcher in innovative equipment and methods, notably in the use of drones to survey areas of the island to collect data on Rapid ‘Ōhi‘a Death and the recent lava flows in lower Puna .

Hart, Perroy, and Morrison worked closely together to develop the detailed methodologies and field schedules. Over two years, the research team combined existing datasets (historic aerial photos) with new data (drone imagery collected by Hart and Perroy) along with topographic surveys to quantify past and present rates of coastal change. These data were then merged with sea level rise projections and other geospatial data to estimate future impacts along the coastline using a Geographic Information System platform.
Also collaborating on the project was Steve Colbert , chair and associate professor of marine science at UH Hilo, and Charles H. “Chip” Fletcher III , associate dean and professor at the School of Ocean and Earth Science and Technology at Mānoa.
Sharing the data
In May 2018, the research team presented their findings to the county planning department, which administers planning regulations for the entire island and provides technical advice to the mayor, planning commission and county council. Based on analyses at Hāpuna, Honoli‘i, and Kapoho, the team offered suggestions for how the county could use scientific data to create place-based setbacks. Predictive models that combined a designated shoreline setback distance, place-based shoreline erosion rates, and structural life expectancy were suggested for calculating setbacks for locations similar to Hāpuna and Honoli‘i. Conversely, elevation-based setbacks were suggested for low-lying coastal communities such as Kapoho.

Throughout the project, the research team worked directly with community members in each of the three study site locations. These interactions were particularly effective along the Hāmākua Coast. The project became so familiar to these communities that it was directly integrated into the Hāmākua Community Development Plan, which called for guidelines for a practical definition of “top of cliff,” a term in shoreline policy that has yet to be clearly defined. Developing common understandings is essential to monitoring and predicting erosion along unstable clifflines that can pose dramatic threats to community infrastructure.
Looking to the future and the uncertainty associated with climate change, the combined results of the completed study will be used to develop policies that are increasingly adaptive to present and future coastal change.
Read the full case study .
Story by Susan Enright, a public information specialist for the Office of the Chancellor and editor of UH Hilo Stories. She received her bachelor of arts in English and certificate in women’s studies from UH Hilo.
Related stories
Climate change research at UH Hilo: Monitoring the coasts for signs of erosion and planning ahead
UH Hilo graduate student wins award for her research using unmanned aerial systems
WATCH: UH Hilo geographer explains his use of innovative drone and mapping technology in his Rapid ʻŌhiʻa Death research

Featured Events

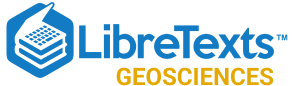
- school Campus Bookshelves
- menu_book Bookshelves
- perm_media Learning Objects
- login Login
- how_to_reg Request Instructor Account
- hub Instructor Commons
- Download Page (PDF)
- Download Full Book (PDF)
- Periodic Table
- Physics Constants
- Scientific Calculator
- Reference & Cite
- Tools expand_more
- Readability
selected template will load here
This action is not available.
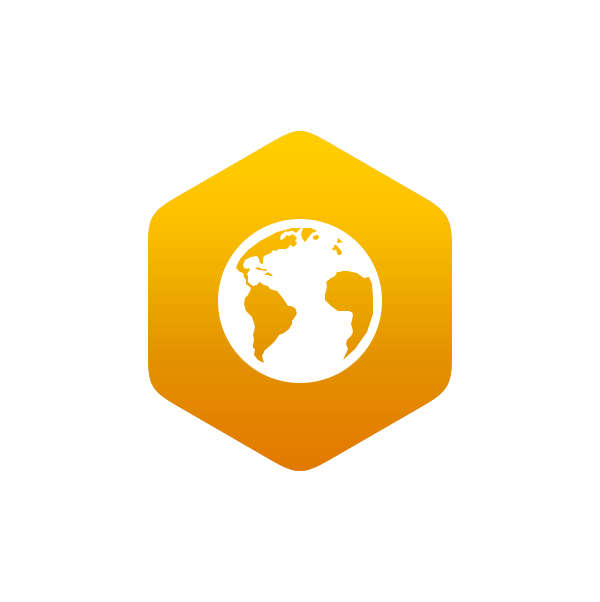
4.2.2: Erosion below the Auxiliary Spillway
- Last updated
- Save as PDF
- Page ID 2809
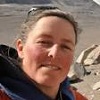
- Dawn Sumner
- University of California, Davis
Image captured from a video taken by drone on February 12, 2017 at 9:30 am: ( Video from the CA Department of Water Resources)
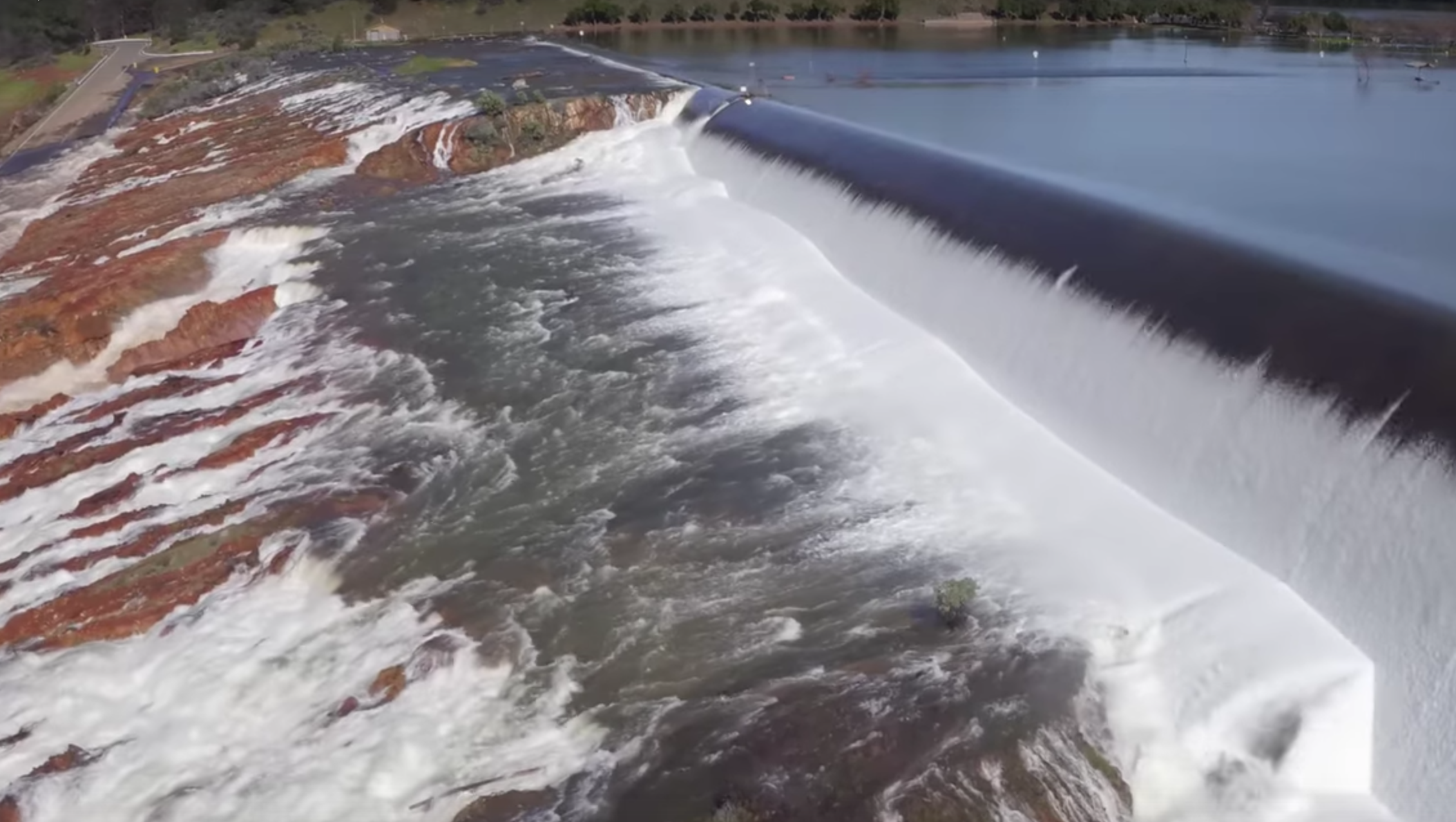
The lake behind the dam is on the upper right, and the spillway extends from the right side of the image to the break in slope near the top. Beyond the break in slope, water is flowing over a parking lot and curb, which it was not designed to do. Note the road in the upper left of the image. Also note that there are no apparent gullies near the base of the spillway, but there is one starting to form near the break in slope.
This image was taken from a different angle at about 3 pm: ( Video from CA DWR)
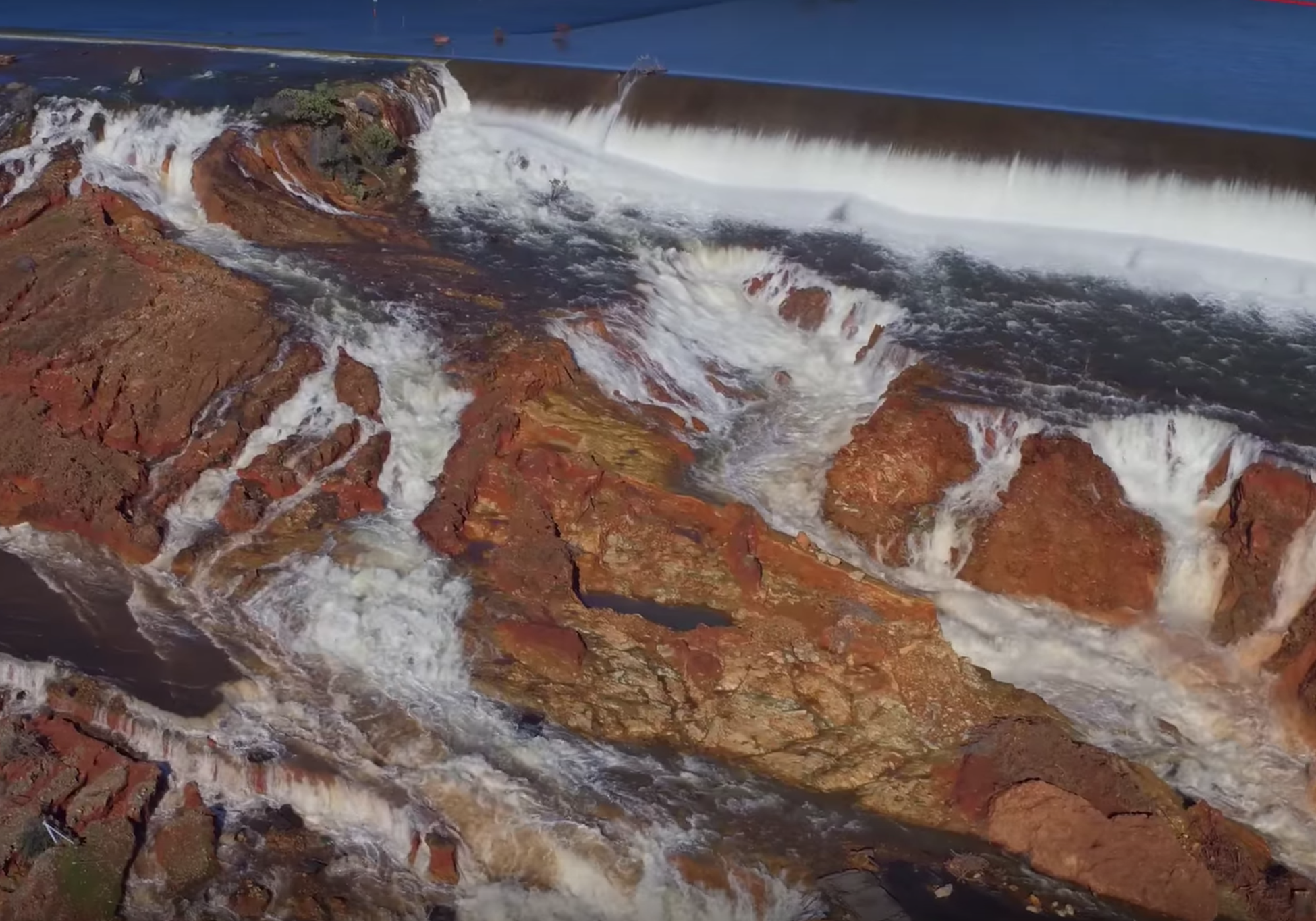
The lake behind the dam is at the top of the image, and the break in slope near the top. Beyond the break in slope is toward the left. The road is under water running across the bottom of the image. Note the prominent gully extending upward toward the spillway near the center of the image. This gully formed between 9:30 am and 3 pm!
Here's what it looked like by 3:30 pm:
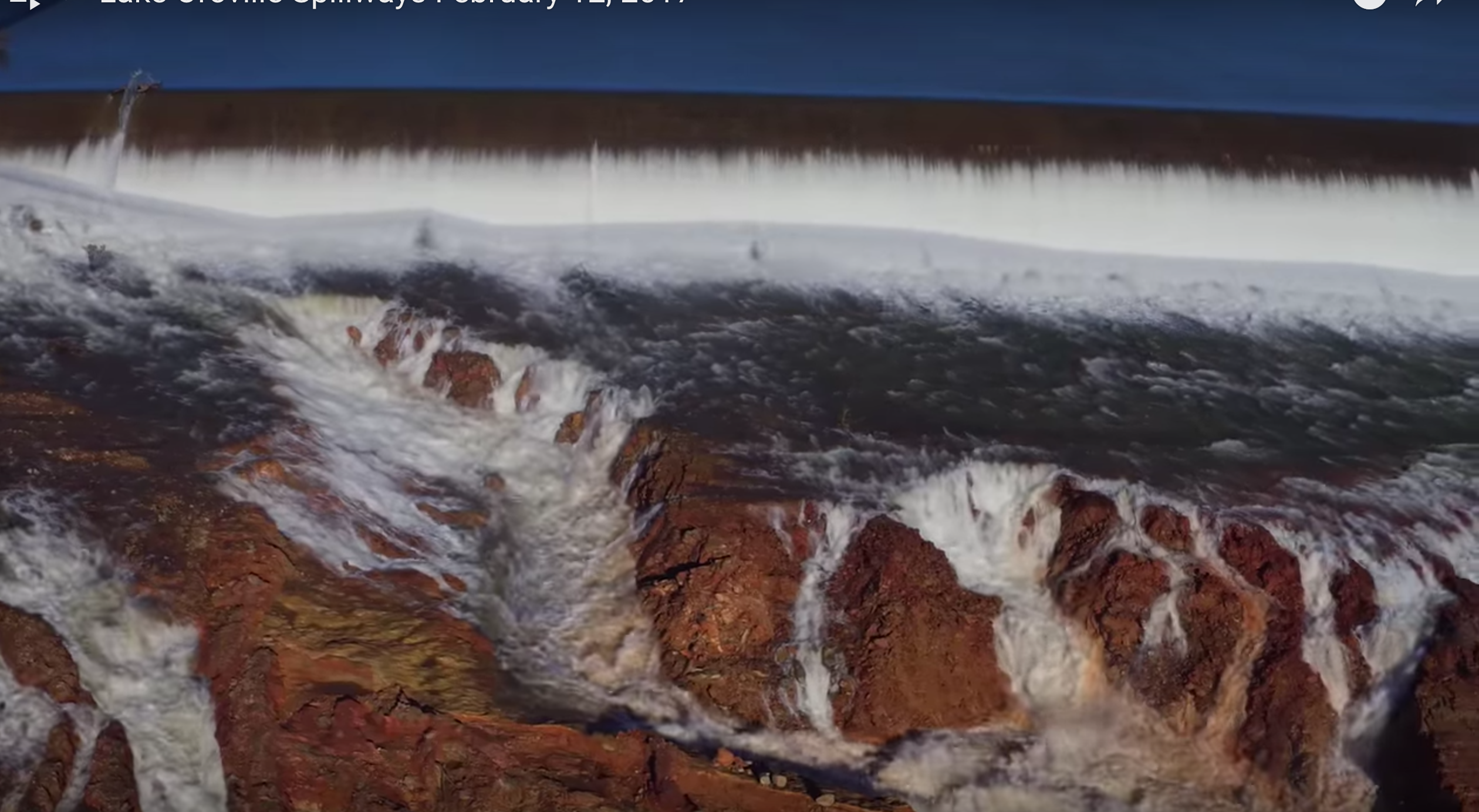
The rapid erosion of the head of this gully triggered emergency management people to decide to evacuate the population. If erosion continued to the spillway and the spillway failed, a 10 m high wall of water might have rushed down the Feather River.
Here is an overhead view after they lowered the water in the dam to below the emergency spillway: (I labeled the "Danger Area".)
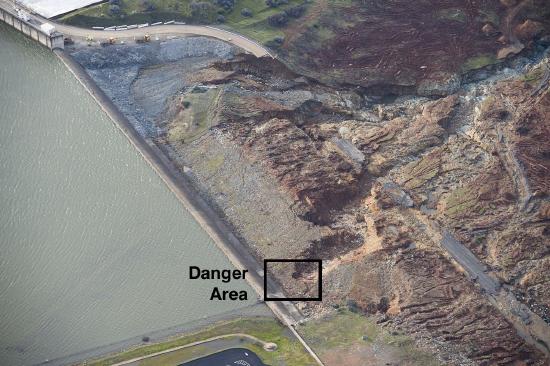
Here's an image of the area from February 16, 2017, showing the progress of engineering work:
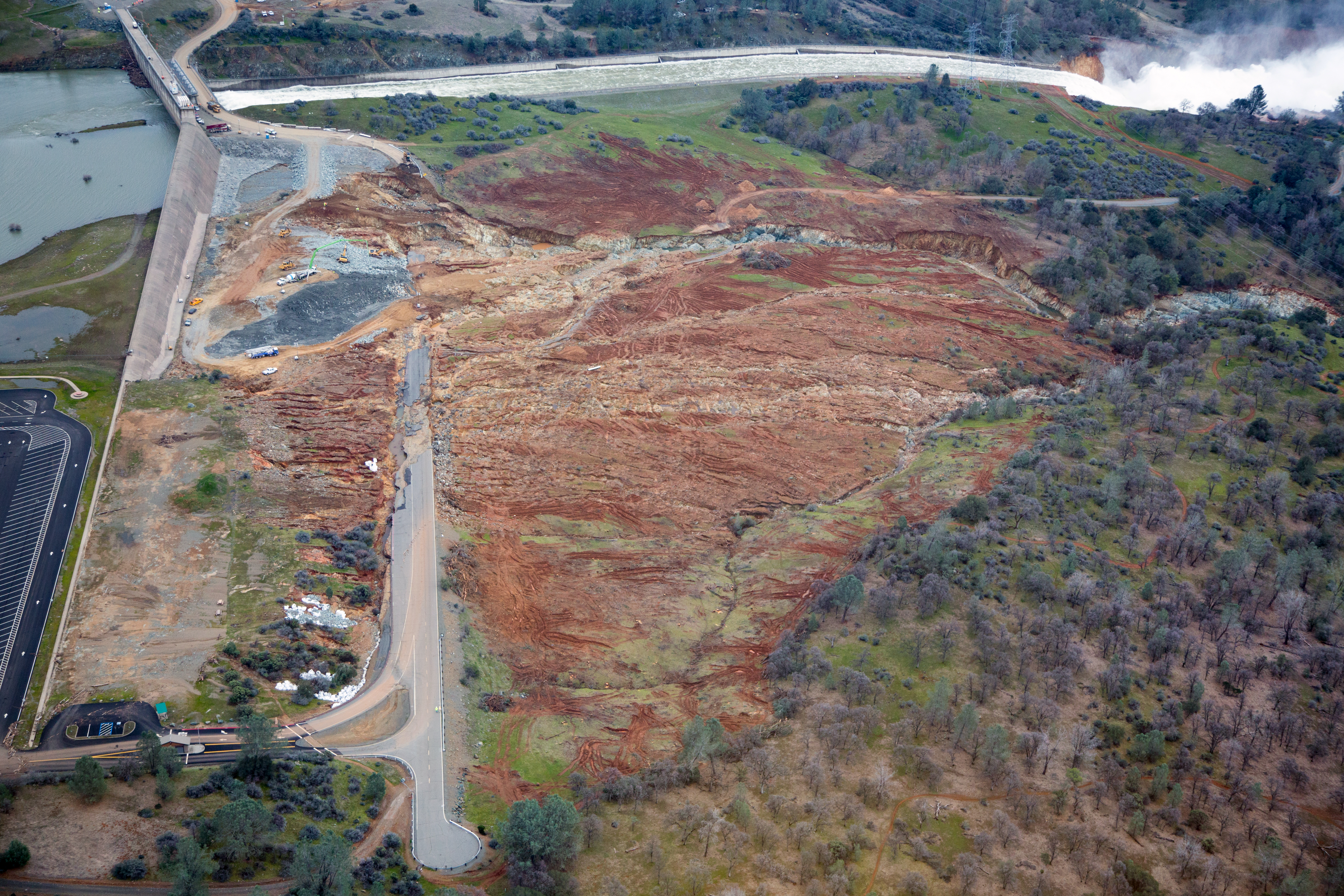
The dark grey area is where the "Danger Area" gully was. Note that it is huge compared to the construction trucks nearby. It has been lined with boulders and concrete. You can see the cement truck and boulders beyond the dark grey area if you expand the image.
- Open supplemental data
- Reference Manager
- Simple TEXT file
People also looked at
Original research article, characteristics and evaluation of coastal erosion vulnerability of typical coast on hainan island.
- 1 Haikou Research Center of Marine Geology, China Geological Survey, Haikou, China
- 2 Third Institute of Oceanography, Ministry of Natural Resources, Xiamen, China
- 3 Fujian Provincial Key Laboratory of Marine Ecological Conservation and Restoration, Xiamen, China
- 4 Key Laboratory of Marine Ecological Conservation and Restoration, Ministry of Natural Resources, Xiamen, China
Coastal erosion vulnerability assessment is widely used to assess the loss degree of coastal zone caused by erosion, and plays an important role in coastal natural resources protection, planning, management and decision-making. Based on the natural and social characteristics of the east coast of Qiongdong and the coastal erosion vulnerability index (CVI) method, this study selected 8 assessment indicators, such as shoreline change rate (U1). The Delphi method and the entropy weight method were used to calculate the comprehensive index weight, combined with CVI method and geographic information system (GIS) technology, to quantitatively evaluate the temporal and spatial distribution characteristics of typical coastal erosion vulnerability such as coral reefs in the east of Hainan Island. The study area was divided into 5 grades: very low (31%), low (10%), moderate (28%), high (24%) and high vulnerability (7%), and the overall performance was moderate erosion vulnerability. The research showed that the interannual downward rate erosion of beach (U3) and the rate of change of the isobath (U2) of the beach were the main controlling factors affecting the vulnerability of coastal erosion in the study area, and the coastal dynamic factor had a greater impact than the other two factors. As a natural barrier, the coral reefs in the study area had good wave absorption and energy reduction, and the coral reef coasts showed low coastal erosion vulnerability, due to the complex hydrodynamic characteristics, estuary coasts is the most vulnerable areas. The verification results of the ROC-AUC method showed that the accuracy of erosion vulnerability was 68.9%, which provided an important reference for the ecological restoration of tropical coral reef biological coasts and the development and management of the Hainan Qiongdong coastal zone.
1. Introduction
The coastal zone is the interaction zone between the ocean and land, and it is also the zone where human activities are concentrated ( Barragan and Andreis, 2015 ). In recent years, with global warming, rapid sea level rise, frequent extreme climate events and intensified human production activities, the coastal zone has been experiencing increasingly serious coastal erosion risks, posing a very large threat to coastal zone engineering, natural ecological coastlines and ecosystems. ( Jones and Phillips, 2011 ; Shi and Kasperson, 2015 ). Coastal erosion is a process of loss caused by the imbalance of sediments in a certain bank section. The main manifestations include shoreline retreat, downward erosion of beaches, high beach stability and low beach erosion, sediment coarsening, etc. ( Mangor et al., 2017 ). Many scholars have studied coastal erosion processes and mechanisms ( Bruun, 1962 ; Chen et al., 1988 ; Chen et al., 2004 ; Thampanya et al., 2006 ), erosion methods ( Cai et al., 2008 ; Cai et al., 2022 ) and model research ( Mohamed et al., 2021 ), erosion hazard evaluation ( Liu, 2015 ), erosion protection management ( Pan et al., 2022 ), and the quantitative relationship between economics and erosion has been systematically studied ( Annelies et al., 2021 ; Hagedoorn et al., 2021 ).
With the in-depth study of coastal erosion, coastal erosion vulnerability assessment is widely used to assess the degree of loss caused by coastal erosion, and it plays an important role in coastal natural resource protection, planning and management decision-making ( Kumar and Kunte, 2012 ; Andrade et al., 2019 ). There are certain differences in the scope, indicators and methods selected by different scholars in the assessment of coastal erosion vulnerability due to differences in coastal regional characteristics. Among them, the evaluation methods include the index method, numerical model method, fuzzy decision method, etc. ( Chu et al., 2005 ). The earliest and most widely used is the coastal vulnerability index (CVI) method proposed by Gronitz ( Gornitz, 1991 ), which comprehensively considers the risk levels of multiple factors, The evaluation index system is established from the aspects of vulnerability characteristics and causes, and the vulnerability index is formed by using mathematical methods to represent the vulnerability degree of the evaluation unit, compared with other methods, This method is simple and easy to operate, and it considers the superposition effect of different index factors, so it is highly scientific. Many scholars use CVI to conduct evaluation research at different levels and scales ( Thieler and Hammar-Klose, 2000 ; Boruff et al., 2005 ; Dominguez et al., 2005 ).
In recent years, scholars have used various additional means to carry out coastal erosion vulnerability assessments, and thus, there has been rapid development and application of coastal erosion vulnerability assessments. Wang et al. (2021) used the percentile ranking method to comprehensively evaluate the physical and social vulnerability of the urban scale in the Yangtze River Delta region, and used bathymetric changes and independent evaluation to verify the reliability of the evaluation results. This method of verifying the results of the assessment is more credible and scientific, and is also the most commonly used method for vulnerability assessments at this stage. Yoo et al. (2014) proposed the environmental vulnerability index (EVI) to evaluate the coastal vulnerability of Jakarta, Indonesia, based on historical disasters and socioeconomic statistics, which were more affected by the vulnerability caused by land-based factors than was the CVI. In addition, some scholars have combined vulnerability, exposure, adaptability and hazard to assess coastal erosion risk ( Merlotto et al., 2016 ; Narra et al., 2017 ; Roy et al., 2021 ; Swami and Parthasarathy, 2021 ). Based on vulnerability research, Swami and Parthasarathy proposed that system vulnerability includes three main factors: exposure, sensitivity and adaptability, which can comprehensively explain and evaluate the inherent characteristics of various coasts. Roy et al. (2021) and others selected 19 indicators, such as physical, environmental and socioeconomic geography, in the Mekhna River estuary area in Bangladesh and used the analytic hierarchy process (AHP) to evaluate the hazard, vulnerability and exposure indices. The area under the curve (AUC) has been used to verify the reliability of evaluating the degree of erosion risk. This research covers 19 index factors and considers almost all the influencing factors that can be involved, making it a more comprehensive assessment of the vulnerability of the Meghna Estuary. Cao Chao ( Cao et al., 2022a ; Cao et al., 2022b ) used different methods to assess the vulnerability of coastal erosion from two scales, the national scale and the small scale of the Pearl River Estuary Greater Bay Area. Furthermore, the innovative introduction of cloud model theory at the national scale to construct a coastal erosion vulnerability assessment system for a comprehensive assessment of vulnerability showed that the cloud model theoretical index system and method were suitable for the assessment of coastal erosion vulnerability in mainland China. Second, on the small scale, a PRE - PRE coastal erosion vulnerability assessment system was established by comprehensively using the AHP, ideal solution similarity ranking technology (TOPSIS), independent weight method, Jenks natural discontinuity method (Jenks), exposure-sensitivity-adaptation (ESA) model and obstacle degree methods. Different evaluation methods were used at two different scales, and good research results were obtained, which made improved the development of research on coastal erosion vulnerability.
At present, scholars have made more assessments of coastal erosion vulnerability, and the vulnerability index factors have mainly selected generalized influencing factors. There is little research on regional typical shore sections, and the individualized and refined considerations of influencing factors are also relatively limited. The differences in the erosion vulnerability of different types of shore sections have not been studied and analyzed. For example, the typical tropical coral reef-type coast, cape bay coast, et al. has not been evaluated for coastal erosion vulnerability, and the difference between different types of coastal erosion vulnerability has not been studied. Based on the tropical coral reef coast, cape bay coast and estuary coast et al. of Hainan Qiongdong, this paper selected the typical coast of Qionghai-Wanning in Hainan Qiongdong. Eight small-scale and typical influencing factors, such as shoreline change rate and shoreline erosion rate, were comprehensively selected from the aspects of coastal morphological characteristics, coastal dynamic characteristics, and socioeconomic and human activities, such as coastline change rate and interannual downward rate erosion. The weight of each evaluation index was determined by the AHP method, Delphi method (DM) and entropy method (TEM). The CVI of the study area was calculated, and the coastal erosion was divided by the vulnerability rating, and a comprehensive assessment of the vulnerability of typical coastal erosion was conducted.
2. Study area
The Qionghai-Wanning coast is located on eastern Hainan Island, China Figure 1A , and is one of the most important coastal development zones for tropical coral reef organisms in China (110°00′~110°40′E, 18°35′~19°29′N) ( Figure 1B ). Its coral reefs are mainly distributed in the nearshore sea area north of Qionghai Tanmen Port, and the type of reefs is fringing reef parallel to the coast. The length of the fringing reef is about 15km from south to north, and the width of the reef flat is about 2km, water depth is about 2m ( Figure 1C ). Quaternary and Cambrian strata are mainly exposed in the coastal zone of the study area ( Tian et al., 2016 ). The climate type is a tropical marine monsoon climate, the tide is an irregular diurnal tide, the wind direction is mainly southerly wind in summer and northeasterly wind in winter, and the waves are mainly mixed waves, among which the mixed waves dominated by swell have the highest frequency, followed by the wind waves, and the pure swell has the lowest frequency. Marine disasters are mainly storm surges caused by tropical cyclones. From 1964 to 2018, a total of 34 typhoons made landfall in the study area, accounting for 35.4% of the total, making the area have the most landed typhoons in Hainan ( Tropical et al., 2021 ).
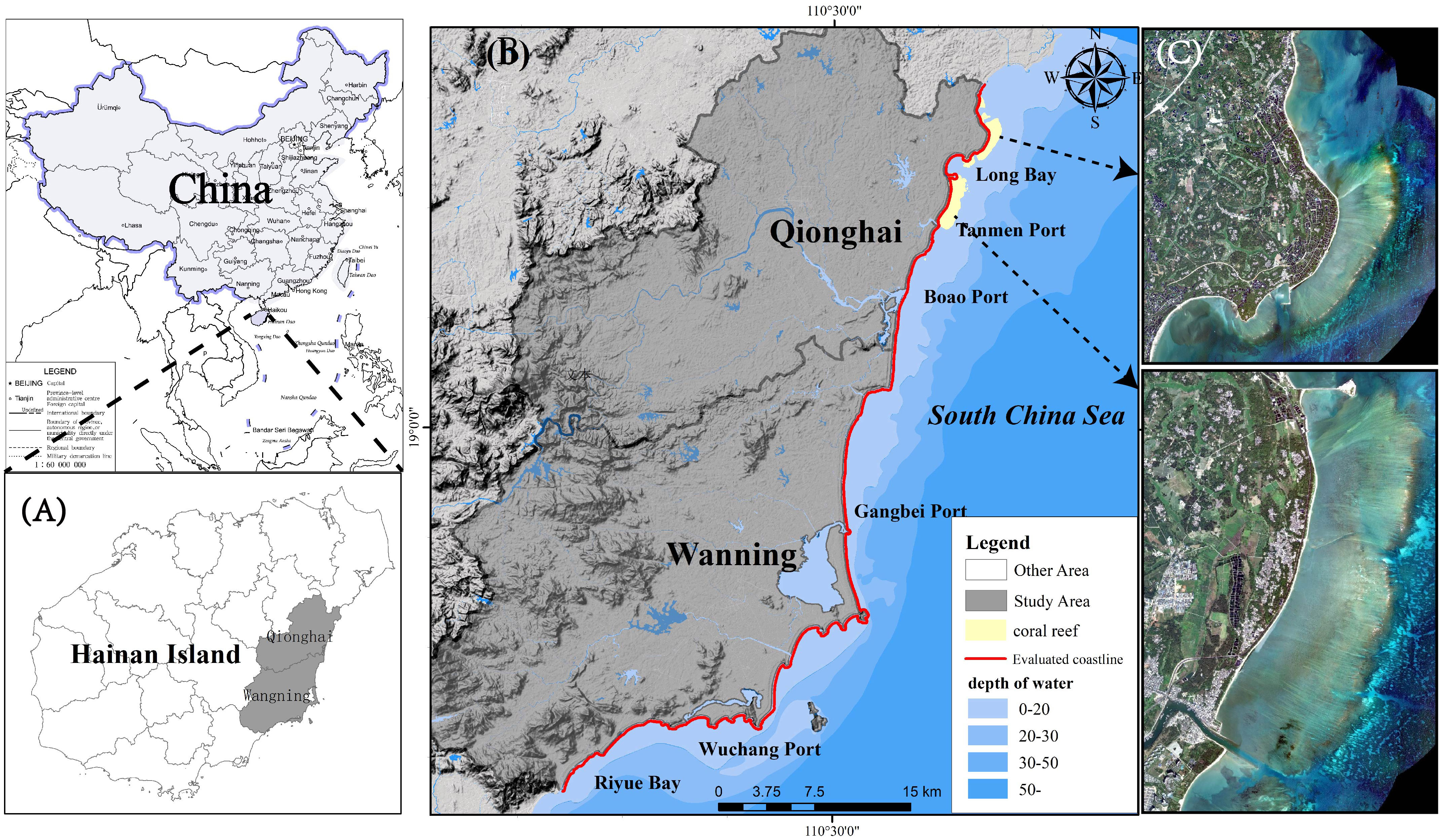
Figure 1 (A) Study area located on the Qionghai-Wanning coast of eastern Hainan (B) Images based on satellite images from China, The assessed coastline is the outer coastline of Qionghai-Wanning. (C) High-resolution remote sensing images of coral fringing reef with a resolution of 0.68 m.
In the 1970s and 1980s, the coastal erosion of Hainan Island already affected approximately 53% of the coastline ( Xia et al., 1993 ), and in the 1990s, the eroded coast of Hainan increased to 71.9%, and there were 64 eroded coasts on the whole island, and erosion was strong. There were 7 shore sections, of which 3 were located in the strongly eroded section of the study area, accounting for approximately 42.8%. Coastal erosion has become the most important disaster problem on the coast of Hainan Island, and the study area is the hardest hit area of coastal erosion ( Chen et al., 2010b ). Research analysis has shown that intensified human activities, such as the construction of reservoirs in the upper reaches of the river, the dredging of riverbeds and coasts, the mining of coral reefs and beach rocks, and various coastal projects, combined with frequent typhoons and other severe marine weather scouring the coast, are the main reasons causing the increasing coastal erosion on Hainan Island ( Ji et al., 2007 ). According to the survey and statistics, the coverage rate of live coral in the study area declined from 32.2% in 2004 to 6.67% in 2018 ( Huang et al., 2019 ), resulting in a great change in the structure of the biological community. Thus, the coast of Qiongdong, Hainan, is suffering from severe coastal erosion.
3. Materials and methods
3.1. establishment of the evaluation index system.
The construction of the evaluation index system comprehensively considers the principles of special regionality, coastal erosion correlation and data extractability and is mainly selected from coastal morphology, coastal dynamics, and socioeconomic and human activity factors. Coastal morphology is the direct bearer of the impact of erosion and is an internal characteristic of the coast, while the dynamic changes in the ocean are the direct external manifestation of the impact of erosion, which is an external featureMattei ( Mattei et al., 2018 ), and socioeconomic and human activities are the driving factors for the occurrence of erosion.
The coastal dynamic characteristics of the coast are reflected from the beach in the supratidal zone, the shoreline in the intertidal zone, and the nearshore waters in the subtidal zone. Intuitive factors, such as the change rate of the shoreline (U1) and the interannual downward rate erosion of the beach (U3), are the most intuitive factors that can be used to characterize the dynamic evolution of the coast. Additionally, the construction of breakwaters, ports and erosion protection walls have prevented the further erosion and retreat of the shoreline to a certain extent, and coastal erosion has shifted into the downward erosion of the underwater bank slope, causing the erosion of the isobath line to approach the shore. Therefore, the rate of change of the isobath (U2) can be used to characterize the erosion of the underwater bank slope. Coastal morphological characteristics are the characteristics of the current state of the coast. Different coastal morphological characteristics bear different degrees of coastal erosion. Coast type (U4), beach width (U5), and beach slope (U6) are the basic components of coastal morphology. Socioeconomic and human activities have a greater impact on the change in coastal appearance, which in turn affects the degree of coastal erosion. Coastal socioeconomics is directly reflected by the degree of coastal development (U7), and the density of human activities (U8), which can indirectly indicate human activities. The evaluation system was constructed by obtaining the index values of each factor from each evaluation unit c1-c29 ( Figure 2A ) ( Cai et al., 2019 ).
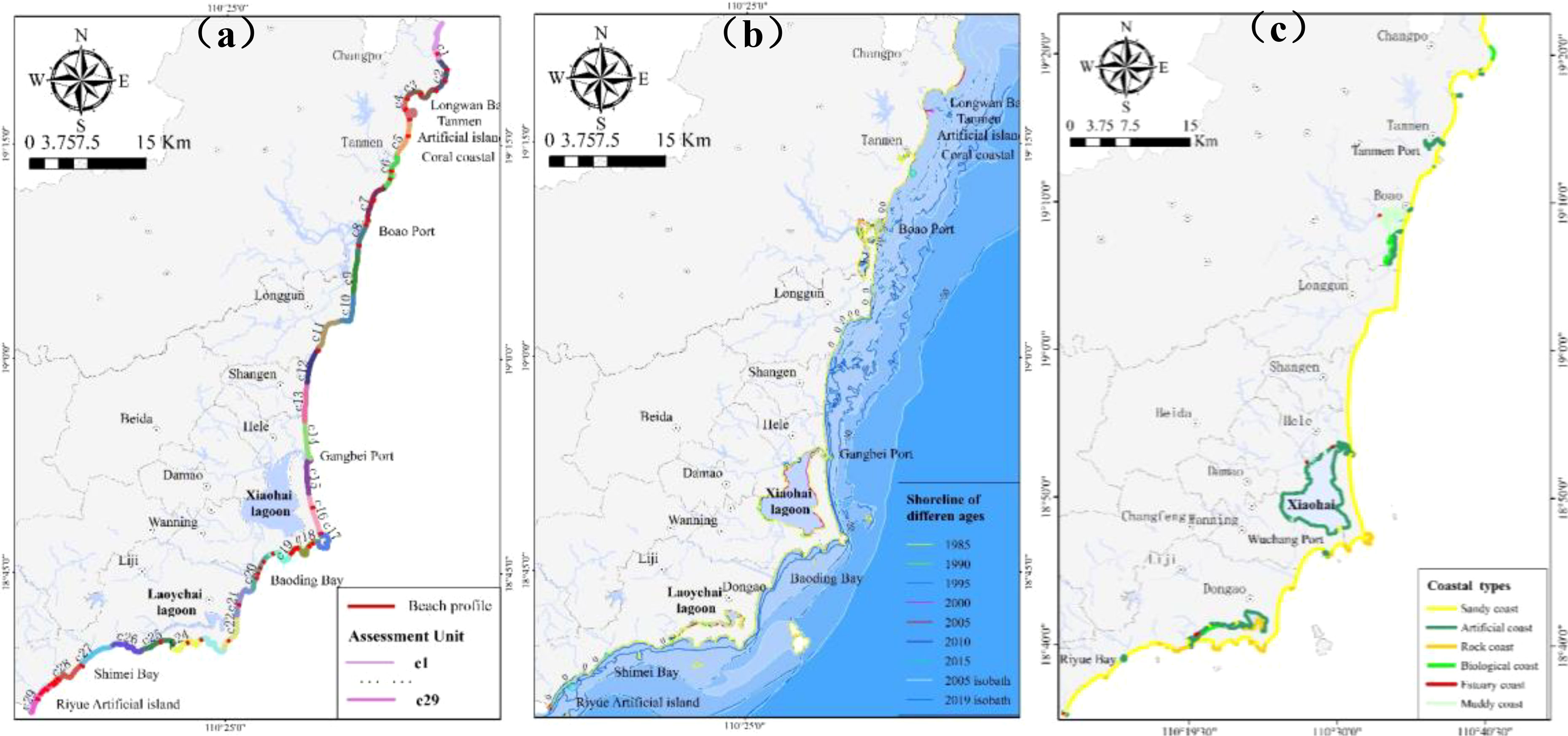
Figure 2 Evaluation factor index element distribution map. (A) Qionghai-Wanning vulnerability assessment unit division map. (B) Distribution map of coastlines in 7 periods from 1985 to 2015 and isobath distribution map from 2005-2019. (C) Qionghai-Wanning 2021 coastline type.
Multispectral remote sensing images are widely used in coastal resource surveys and dynamic evolution studies, and for coastline extraction, they have the advantages of large-scale and rich spectral features ( Boak and Turner, 2005 ). To reduce the interpretation errors caused by remote sensing images of different resolutions and different time periods, this paper adopted Google Earth satellite images with the same resolution and the same season and selected the years 1985, 1990, 1995, 2000, 2005, 2010 and 2015, for a total of 7 time periods of remote sensing image data ( Figure 2B ), with a resolution of 16.8 m. Visual interpretation was used to extract the coastlines of each year based on the waterline ( Alberico et al., 2012 ; Yang, 2013 ). The shoreline change rate adopted the LRR method provided by the digital coastline analysis system DSAS4.3 ( http://woodshole.er.usgs.gov/project-pages/dsas/ ) ( Deepika et al., 2013 ), starting from the northernmost part of the Qionghai administrative boundary. At the starting point (110°40’15” E, 19°21’32” N), a section was generated every 50 m, and a total of 2,910 cross-sections were used to analyze the changes in the Qionghai-Wanning coastline. The calculated value was negative, indicating that the coast was eroded, while a positive value would indicate that the coast was silted up ( Table 1 ).
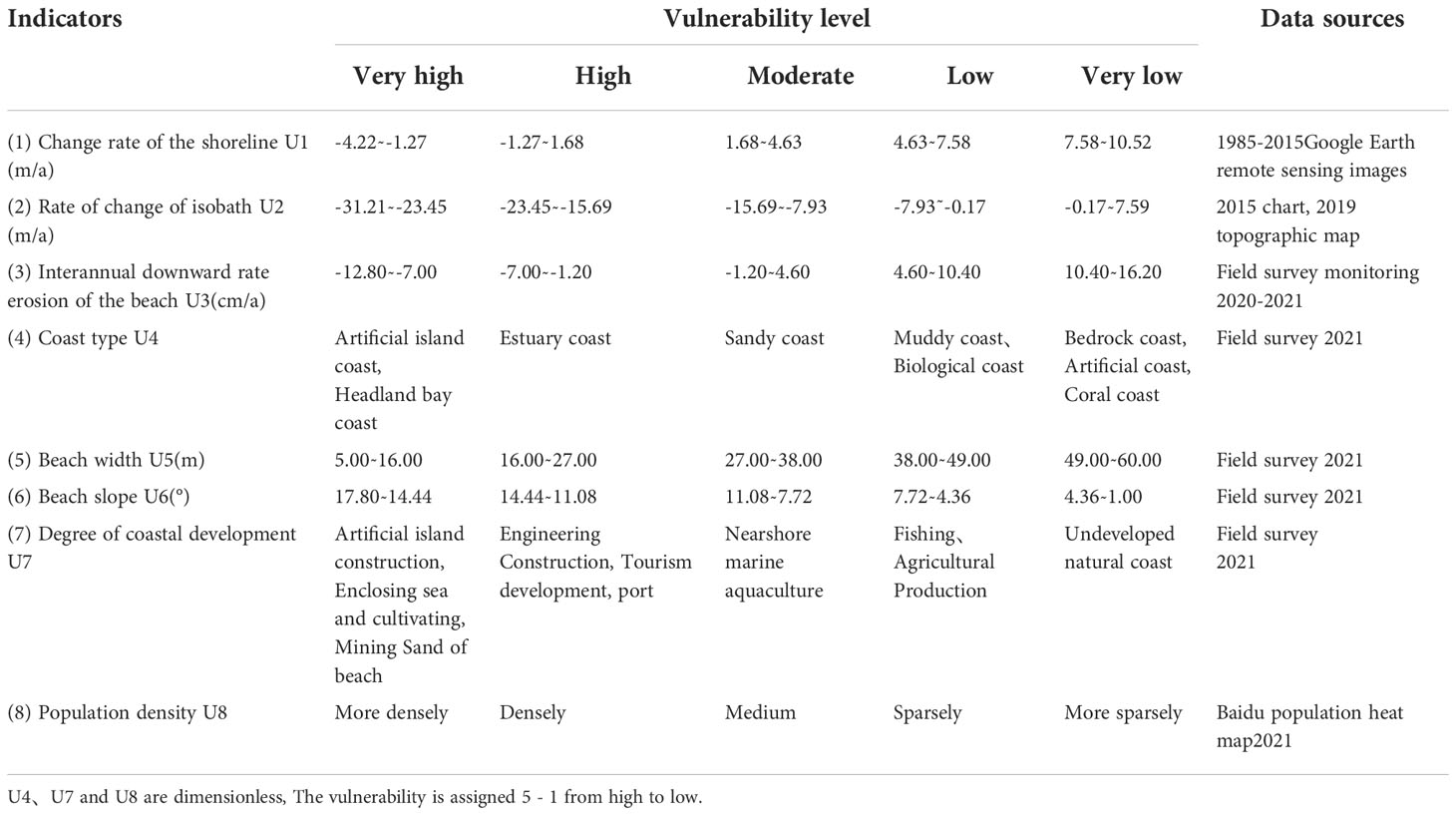
Table 1 Assessment indicator vulnerability grading scale.
We compared and analyzed the erosion and deposition of the shallow seabed by collecting multiple periods of charts or isobath data in topographic maps. This paper used the 2005 nautical chart and the 2019 1:50,000 topographic map isobath 2 data ( Figure 2B ), additionally, the LRR method in the DSAS plug-in was used to calculate the 20-m isobath changes from 2005-2019 ( Table 1 ).
The direct manifestation of coastal erosion is that in addition to shoreline retreat, downward rate erosion of beaches is an important manifestation and a critical factor in vulnerability assessment. However, at present, many scholars have not considered and analyzed the vertical erosion and siltation changes of the beach due to the large assessment scope and the lack of long-term continuous monitoring, which makes the vulnerability assessment results unrepresentative. In this paper, the monitoring data of 44 beaches in the Qionghai-Wanning coastal zone were used to calculate the interannual erosion and silting rate of each beach through interannual monitoring. If there was no beach, such as bedrock and artificial shoreline, the erosion rate of the beach was 0. This is the first assessment to incorporate the interannual downward rate erosion of beaches into the vulnerability assessment system, making the assessment results more scientific and representative ( Table 1 ).
The coast itself is the direct bearer of coastal erosion, the study area has typical coral reef coast, cape bay coast, estuary coast, artificial island coast, etc. and different coast types have different degrees of erosion vulnerability. Such as coral reef coast, A large amount of coral reef debris is washed and deposited on the bank by the tide to form a coral reef beach ( Figure 3A ), which can dissipate wave energy and protect the beach from erosion. At the same time, the length, width, coarse rate and water depth of the fringing reef also have a great effect on wave energy dissipation, therefore, the coral reef coast has a low degree of vulnerability. In addition, the corresponding erosion vulnerability of coastal types, such as estuaries, artificial islands, and ports, presents different states ( Figures 3B, C ). The coast type data come from the field survey measurement data of the study area in 2021 ( Figure 2C ). Since the coastline type is dimensionless, the weights of erosion and siltation effects are assigned according to different coastline types ( Table 1 ).
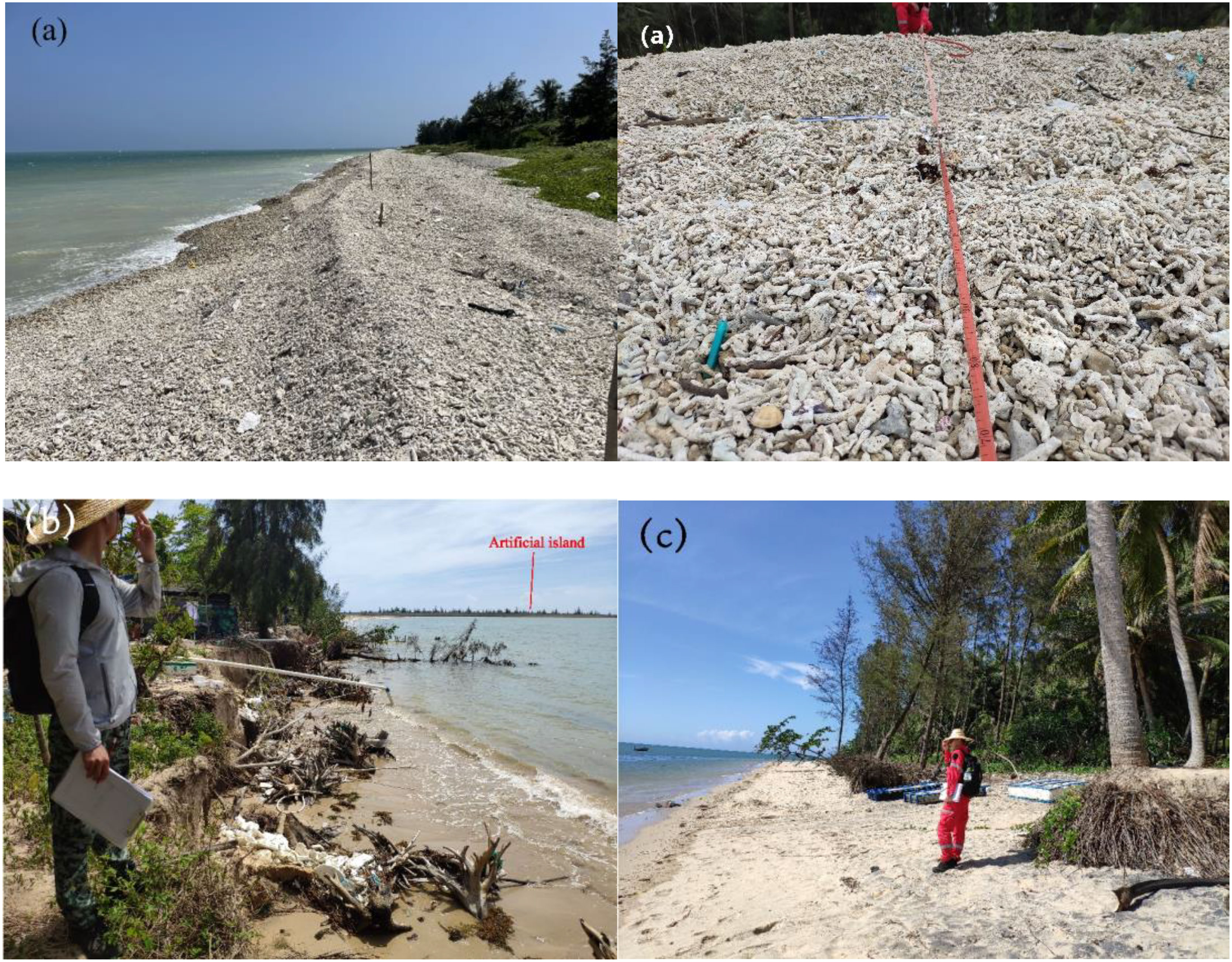
Figure 3 (A) In coral reef shores, coral detritus is washed ashore and deposited to form a reef beach. (B) Coastal erosion scarps on artificial island coast. (C) Straight shore erosion.
The beach width is characterized by the distance between the water edge and the grass edge or the shelterbelt; the smaller the width of the beach is, the greater the energy of tidal water scouring the beach, the higher the degree of erosion and retreat of the corresponding shoreline, and the higher the vulnerability. Wider beaches often fail to scour vegetation slopes and have a larger distance for buffering and releasing energy, so their vulnerability is relatively low. The beach width data in this paper were obtained from a field survey of the field research area in 2021 ( Table 1 ).
The greater the slope value of the beach is, the stronger the scouring ability of the waves to increase water and climb the slope, and the corresponding erosion vulnerability is larger and vice versa. The beach slope data were derived from field survey measurement data in the study area in 2021. If there were several survey points in a unit, the average value of the beach slope recorded at each survey point was taken as the slope value of the section ( Table 1 ).
The socioeconomic development and construction of coastal areas also enhances the degree of vulnerability to coastal disasters, making the degree of coastal development an indispensable factor in vulnerability assessment ( Dolan and Walker, 2006 ; Bathi and Das, 2016 ; Hoque et al., 2019 ; Alam et al., 2020 ). The degree of coastal development mainly refers to the status quo of coastal utilization and development, including tourism development, aquaculture development, port and dam construction, man-made engineering construction and development. The higher the degree of development is, the higher the corresponding vulnerability index ( Table 1 ).
The density of human activities is represented by the density of human activities on different coasts in the same time period or in a specific time period. The rapid growth of coastal populations and changes in natural habitats have brought greater pressure on coastal land, and the degree of vulnerability has gradually increased; in contrast, natural coasts with less human activities have better coastal beaches and environments, and their vulnerability is lower ( Parvin et al., 2008 ; Appeaning, 2013 ; Narra et al., 2019 ). There are different anthropogenic activities, such as fishing activities, tourism, and resident population activities, along the coast of the study area. The data were obtained through the Baidu population heatmap to obtain the relative activity data of the Qionghai-Wanning coastal population in the same time period to characterize the anthropogenic activity density (accuracy is 1 km) and assign values to different personnel densities ( Table 1 ).
3.2. Index weights and evaluation methods
In a multi-indicator evaluation decision, it is necessary to prioritize indicators and find the advantage or weight level of each indicator relative to other indicators to achieve a more accurate evaluation goal. Various commonly used methods in verifying effectiveness include the AHP ( Mosadeghi et al., 2015 ; Cabrera and Lee, 2019 ; Saffaria et al., 2020 ), maximum entropy model (MAXENT) ( Cabrera and Lee, 2020 ), rank weight method ( Alam et al., 2020 ), proportional weight method ( Dou et al., 2017 ), fuzzy analytic hierarchy process method ( Wijitkosum and Sriburi, 2019 ), and fuzzy logic method ( Hoque et al., 2021a ), and Cabrera and Lee showed that the AHP method was a reliable method in vulnerability assessment research based on multiple indicators. In this study, a combination of DM and TEM methods was used to analyze and calculate the index weights.
(i) In the DM method, the consistency ratio ( CR ) was introduced as a reliable index for judging the consistency of the matrix to overcome the randomness in the index judgment process ( Saaty, 1980 ). The CR represents the comparison between the consistency index ( CI ) and the random consistency index ( RI ). When CR<0.1, the corresponding judgment matrix is acceptable for the AHP method, and if CR >0.1, the judgment matrix is readjusted.
The calculation formula of DM is as follows:
(1) where λ max is the largest eigenvalue of the n -order judgment matrix, n is the number of evaluation indicators. (2) where λ ¯ m a x is the average value of the maximum eigenvalues of the n -order random positive and negative square matrix.
The TEM calculation formula is as follows:
Where constant k =1/ln(n) in (4), m is the number of factor indicators, n is the number of evaluation units. 0≤ a i ≤ 1, ∑ i = 1 m a i =1.
The comprehensive weight values of the indicators calculated based on the DM and TEM are shown in Table 2 .
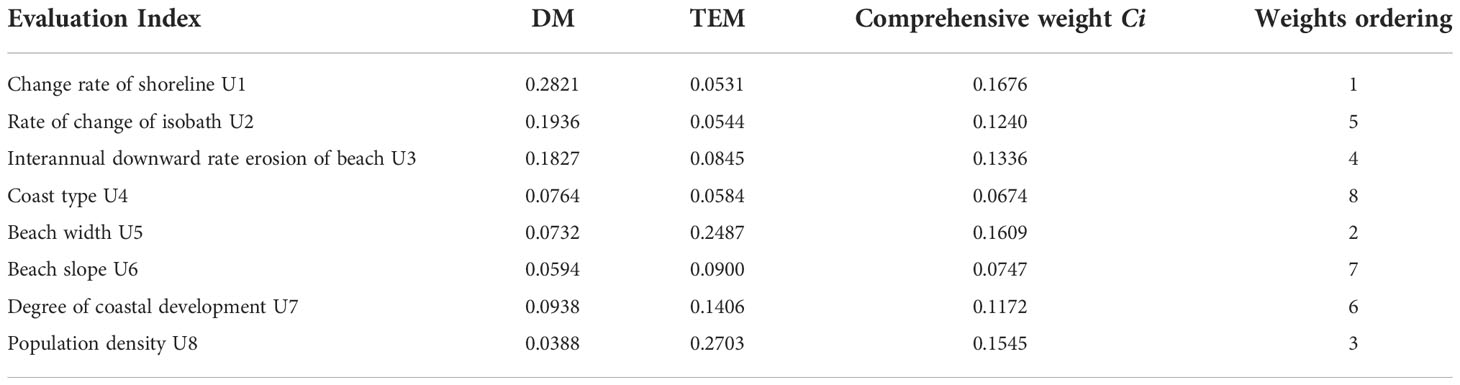
Table 2 Index weight value of coastal erosion vulnerability assessment.
The CVI has two mathematical calculation methods: one is to find the square root of the “product”, and the other is to weight the “sum”. When the weight of each indicator can be determined, the weighted summation method is adopted ( Liu et al., 2013 ), and the formula is as follows:
In the (7), P i is the quantitative grading value of each evaluation index, and C i is the weight value corresponding to the index.
4.1. Temporal and spatial distribution characteristics of erosion and siltation
4.1.1. spatial distribution characteristics of erosion and siltation.
In 2021, there were 37 eroded shore sections and 5 silted shore sections in the study area. The longest eroded section was located in Baoding Bay, south of Wanning Wuchang Port, with a length of 3.5 km, and the shortest was 37 m ( Figure 4 ). The cumulative length of the eroded section in Qionghai-Wanning was approximately 26.12 km, accounting for approximately 17.96% of the total shore section. The accumulative length of the silted section was approximately 4.95 km, accounting for approximately 3.40% of the total shore section, and the stable section accounted for approximately 78.64% of the total shore section. The eroded shores were widely distributed and mostly occurred on the northern and southern coasts of the artificial island, as well as in headland bays, both sides of the estuary, and seaward protruding shores. Among them, erosion must occur on the coasts of headlands and artificial islands, and the degree of erosion was significantly stronger than that of the straight coast. The siltation section was less distributed and occurred in the inner side of artificial islands, in the angle between the harbor and the dam, and on the floodplain position of the estuary.
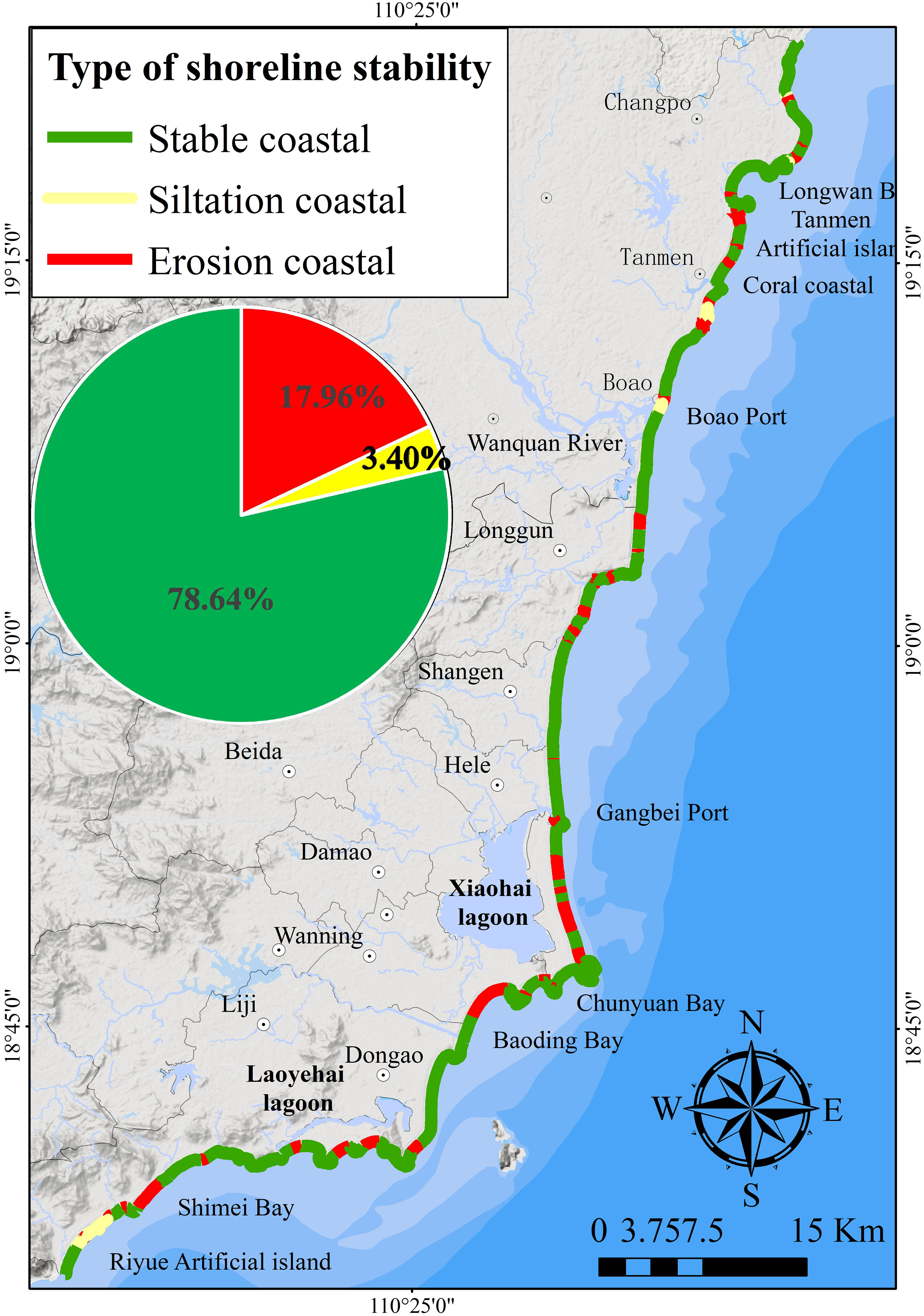
Figure 4 Spatial distribution map of coastal erosion and deposition in Qionghai-Wanning in 2021.
The monitoring results of the beach profile showed that most of the beaches are in a state of erosion, and the shore sections with relatively large downward erosion were mainly distributed in artificial islands, headlands and estuaries, such as Qionghai Longwan, Tanmen Artificial Island, Coral Island, and Riyue Island in Wanning. The maximum downward erosion rate was located at the jade belt beach of Wanquan Estuary, with a value of approximately -12.8 cm/a, followed by that in Riyue Bay in Wanning, showing a rate of decline of -10 cm/a.
4.1.2. Temporal distribution characteristics of erosion and siltation
The most direct characterization of coastal erosion and siltation is the advance and retreat of the coastline. From 1985 to 2015, 7 periods of remote sensing images were used to monitor the rate of change of the coastline and showed that ( Figure 5 ), the Qionghai-Wanning coastal was in a state of weak siltation and erosion as a whole, and it was distributed throughout the northern and southern coasts of Qionghai-Wanning. Among them, the siltation rate of 0-6.29 m/a accounted for the largest proportion, approximately 50.15%, and was mainly distributed in the relatively straight sandy section, such as Gangbei port in the north section and the Wanquan River mouth in the north and south sections. Second, the weakest erosion rate was -0.99-0 m/a, accounting for approximately 39.54%, and these areas were mainly distributed along the coast of Shimei Bay, Riyue Bay and other cape bays in Wanning. The maximum erosion rate was -1.00~-7.58 m/a, accounting for approximately 6.77%, and these areas were mainly distributed in the Wanquan River estuary and the Tanmen artificial island section. The maximum rate of siltation on the coastline was 20.74-39.49 m/a, accounting for approximately 0.65%, and these sites were mainly north of Longwan Port. From the 1990s to the early 2000s, villagers reclaimed and built ponds in the shallow coral reef area and continuously reclaimed them to the sea, making the coastline advance to the sea at an increasing rate. Moreover, the construction of impervious roads connecting the artificial islands to the islands increased the artificial coastline, which also had a significant impact on coastal erosion and siltation.
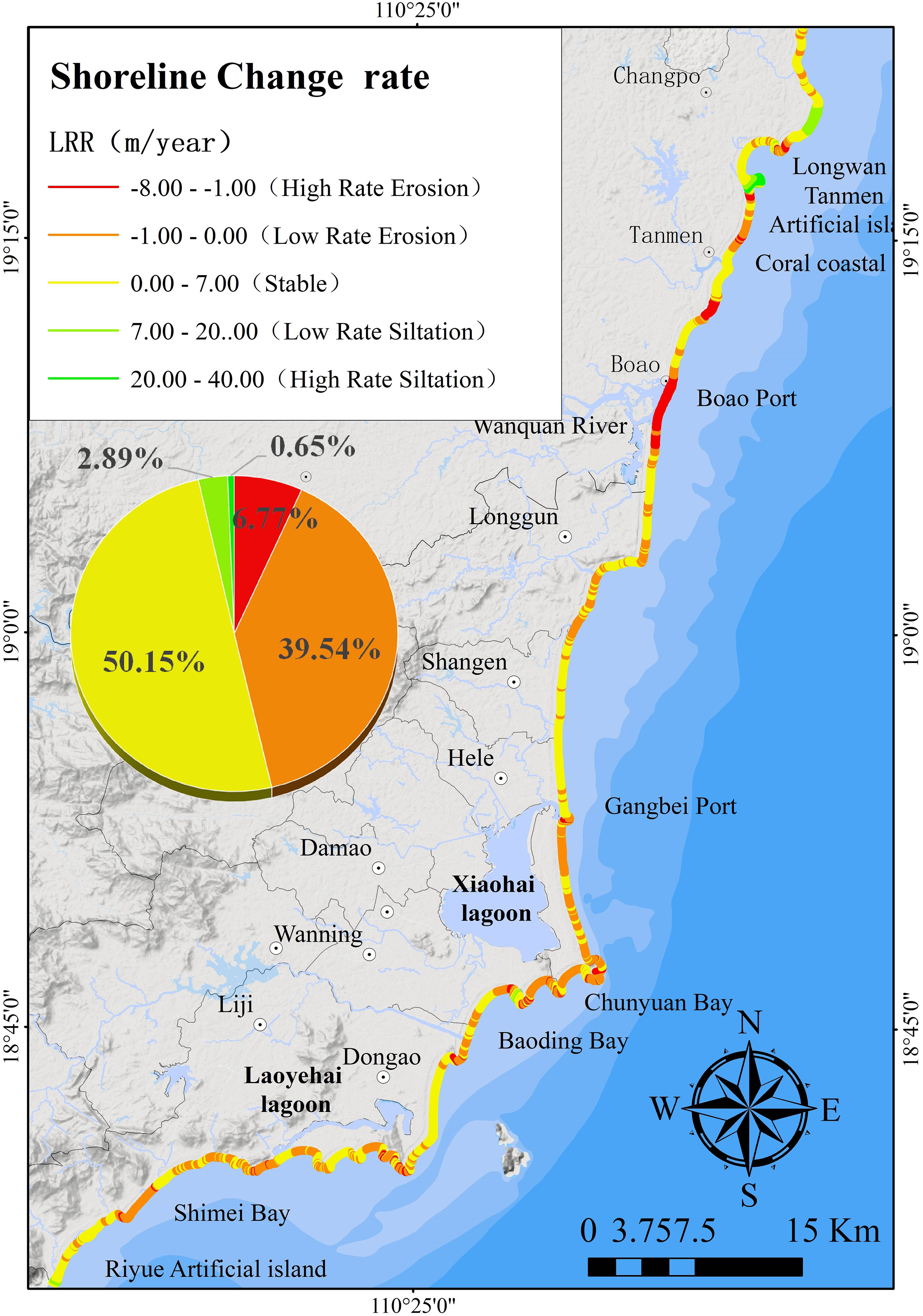
Figure 5 Distribution characteristics and proportion of Qionghai-Wanning coastline change rate from 1985 to 2015.
4.2. Characteristics of coastal erosion vulnerability
4.2.1. spatial distribution characteristics of coastal erosion vulnerability.
The erosion vulnerability assessment results are shown in Figure 6 . Most of the coastal erosion in Qionghai-Wanning is in a state of moderate vulnerability or above, accounting for approximately 59% of the total shore section, of which the vulnerability of high and above exceeds 31%, including 2 with very high vulnerability and 7 with high vulnerability. The very high vulnerability was distributed in the Wanquan Estuary of Qionghai and the nearby coasts to the south. The high vulnerability was distributed in some capes and estuaries, such as to the south of the Wanquan estuary and on the nearby shores of Wanning Shimei Bay, while a small part of the straight coasts had a higher vulnerability status. 8 locations had moderate vulnerability, accounting for approximately 28%, and the sites were relatively scattered, mainly distributed in some inner bays of the headlands, such as Wanning Dongwo Bay and Shimei Bay. There were 12 places with low vulnerability and below, including 3 places with low vulnerability and 9 places with very low vulnerability, accounting for approximately 41% of the Qionghai-Wanning coast, and these sites were mainly distributed in the coral reef area to the north of Tanmen Port, the headlands formed by various bedrocks, such as Wanning Dahuajiao, Shimeiwan headland, and the coasts where bedrock and sand alternately appear. Areas with hard engineering, such as port terminals and damp-proof gates also had a lower vulnerability.
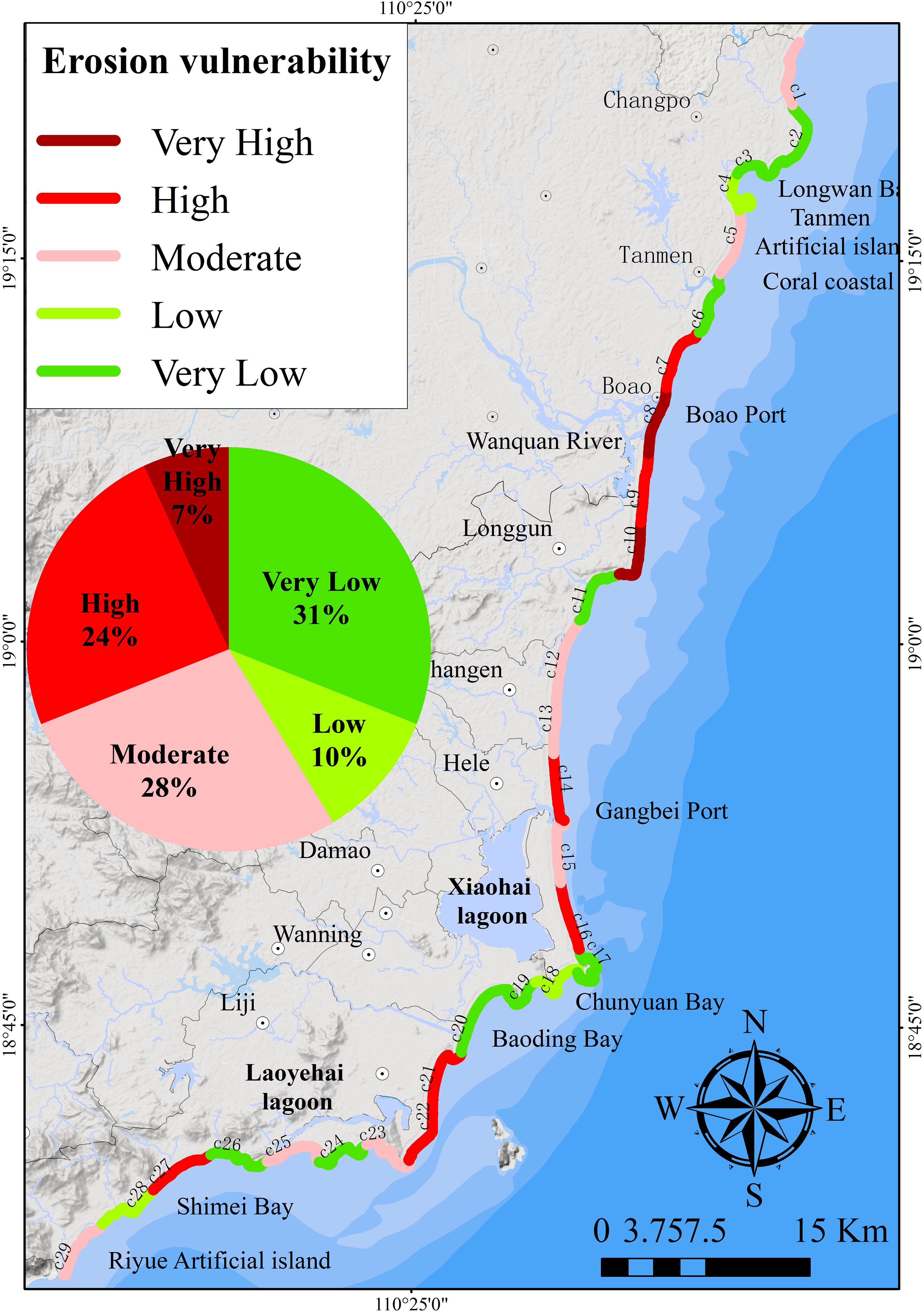
Figure 6 Spatial distribution characteristics and proportion of coastal erosion vulnerability in Qionghai-Wanning.
4.2.2. Vulnerability level characteristics
4.2.2.1. very high vulnerability.
The very high vulnerability of Qionghai-Wanning coastal erosion occurred in the C8 and C10 sections, located in the Wanquan River mouth and adjacent coasts, which corresponded to the erosion intensity of the Wanquan Estuary. The interannual downward erosion rate U3 of the beach in this section was approximately -12.8 cm/a, which was the maximum value in the study area ( Figure 7A . U3). This was related to the complex and strong hydrodynamic effects of the estuary. In addition, the beach slope (U6) of this section was generally higher than that of the other sections, and anthropogenic activity (U8) had a higher value due to the influence of intensive human activities such as Boao tourism and boat navigation. In general, the very high vulnerability was due to the dominant influence of a higher interannual downward erosion rate, steeper beach slope and denser anthropogenic activities.
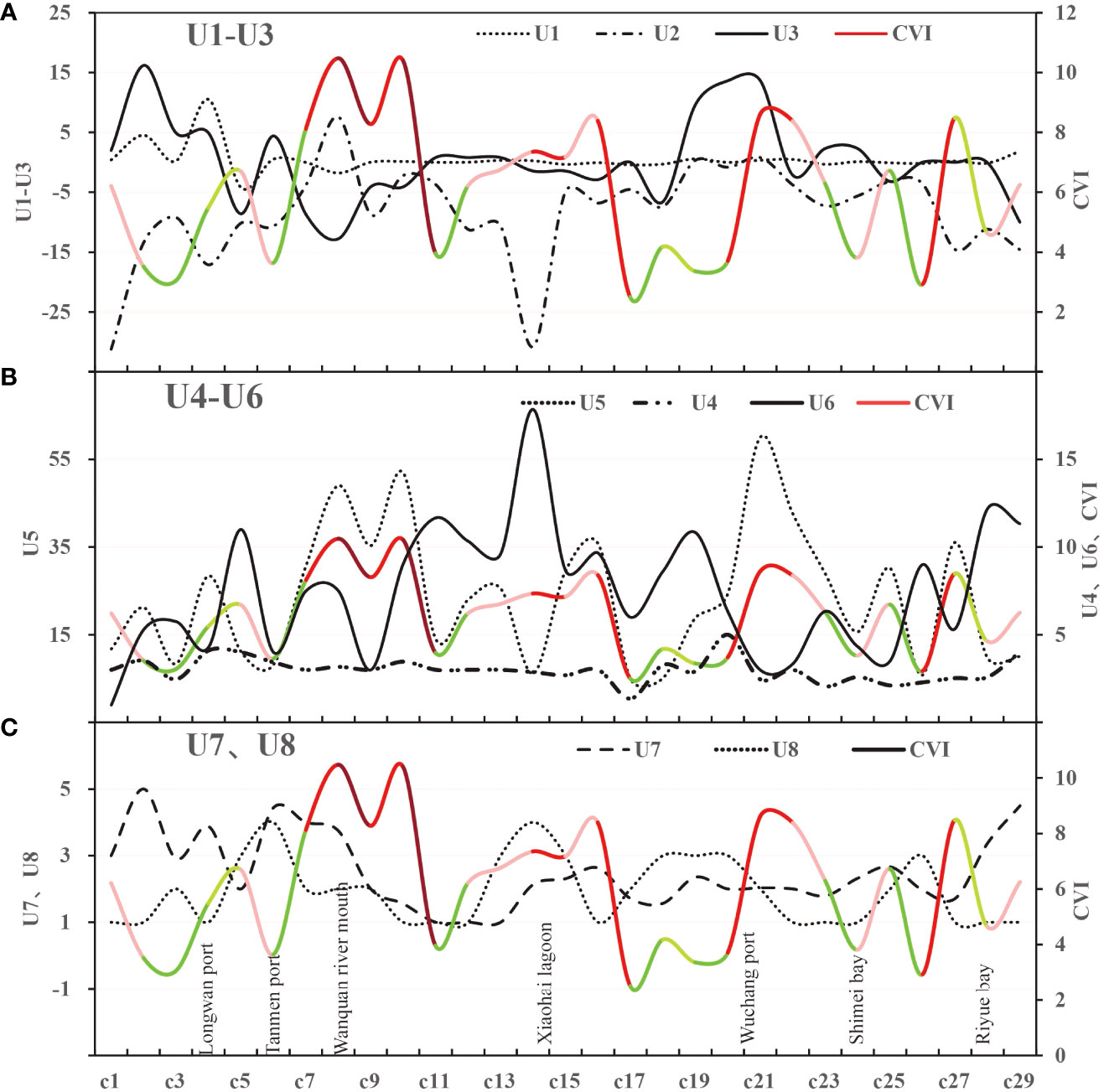
Figure 7 Vulnerability index and evaluation factor curve (The color of CVI curve corresponds to its vulnerability degree). (A) The relationship between U1-U3 index and CVI curve. (B) The relationship between U4-U6 index and CVI curve. (C) The relationship between U7, U8 index and CVI curve.
4.2.2.2. High vulnerability
The areas with higher vulnerability were mainly distributed along some coasts, such as the headland bays and estuaries of northern and southern Wanquan estuary (C7) and the coastal sections near Shimei Bay (C27) in Wanning. The section with a high vulnerability distribution was accompanied by an obvious erosion phenomenon, and the beach slope (U6) of the high vulnerability c14 section had a maximum slope of approximately 65° ( Figure 7B . U6). The overall slope range was generally 7-11°, and the interannual downward erosion rate (U3) of the beach was between 0 and 1.2 cm. It had obvious characteristics, such as more human activities; in addition, the coasts with high vulnerability had the characteristics of wider beaches, and the other factors had no obvious common characteristics. In summary, high vulnerability was characterized by a certain width of beach, and the slope and human activities had a greater impact.
4.2.2.3. Moderate vulnerability
The Qionghai-Wanning moderate vulnerability distribution was relatively scattered, and it was moderately vulnerable in the straight shore section, some headland coasts, and coral reef coasts. Additionally, we found that under the protection of coral reefs, the degree of vulnerability was generally low, but under the combined influence of artificial islands and coral reefs, the degree of vulnerability increased on artificial island coasts. For example, section c5 in the Tanmen artificial island area was moderately vulnerable. The common feature of moderate vulnerability was that the isobath change rate (U2) was between -15.69 and -7.93 m/a, and the rest had no obvious features.
4.2.2.4. Low and very low vulnerability
Except for the low vulnerability of some coral reef shore sections, bedrock shorelines, hard artificial shorelines, etc., the remaining low-vulnerability sections had obvious common characteristics, such as relatively gentle bank slopes, lower shoreline change rates, smaller isobath change rates, and interannual siltation states of beaches ( Figure 7 ). Furthermore, some beaches had better protection under the action of alternate bedrocks and showed lower vulnerability; therefore, the hard coast type played a leading role in lower vulnerability.
5. Discussion
5.1. variation in coastal erosion vulnerability.
Through the Spearman correlation coefficient method ( Rodgers and Nicewander, 1988 ), statistical analysis was carried out on the correlation between the raw data of each evaluation factor and the vulnerability index, and the results are shown in Table 3 .
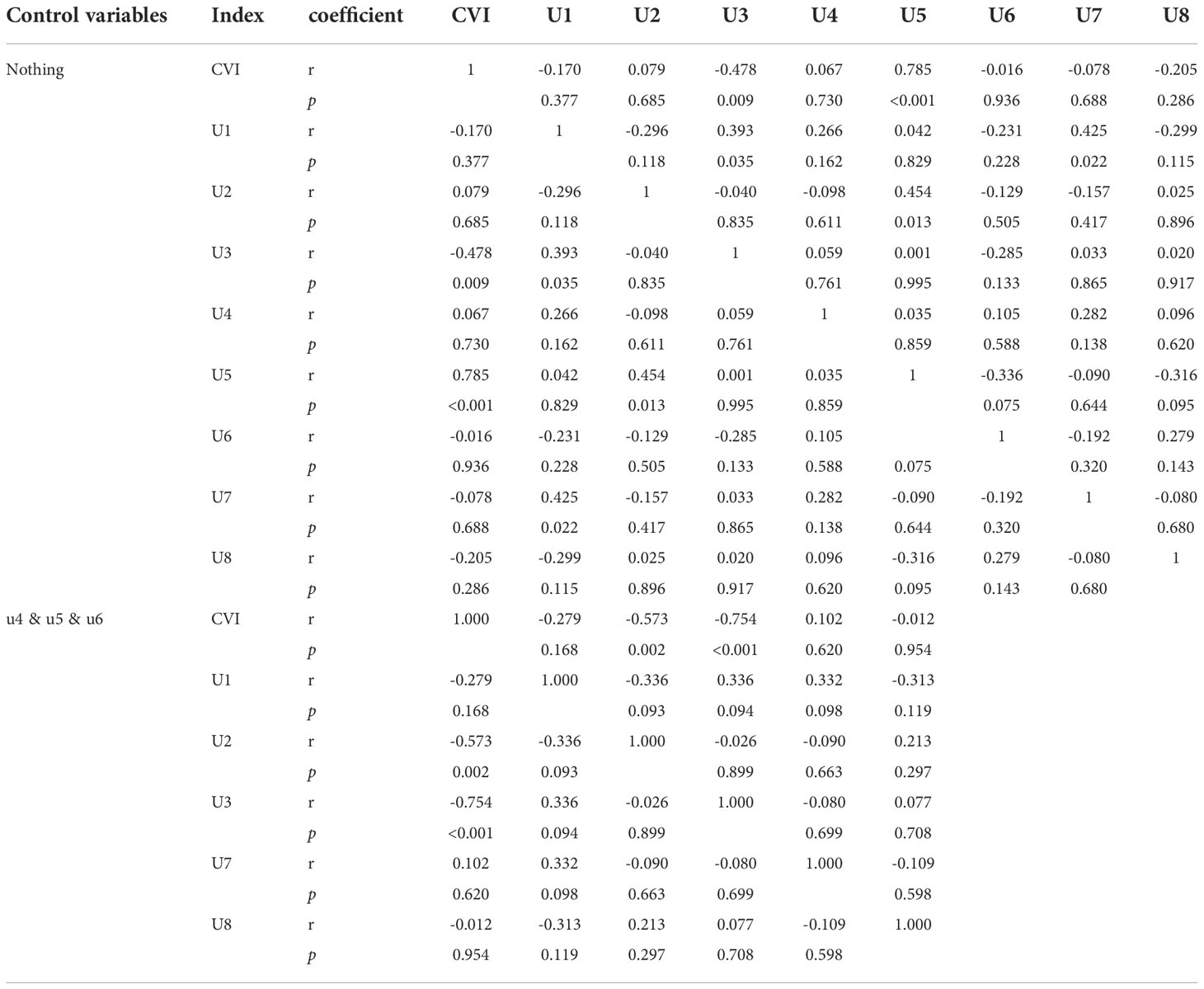
Table 3 Correlation between CVI and each evaluation factor based on Spearson method.
It was assumed that there was no mutual influence between the indicators; that is, in the absence of control variables, the interannual downward rate of erosion of beaches (U3) was moderately negatively correlated with the CVI ( r =-0.48, p <0.01), which showed that the greater the rate of downward erosion was, the greater the degree of vulnerability. The beach width (U5) was strongly correlated with the CVI ( r =0.79, p <0.01) ( Figure 8 ), which indicated that the beach wider had a greater degree of vulnerability. The other indicators were not significantly correlated with the CVI ( |r| <0.3, p >0.05).
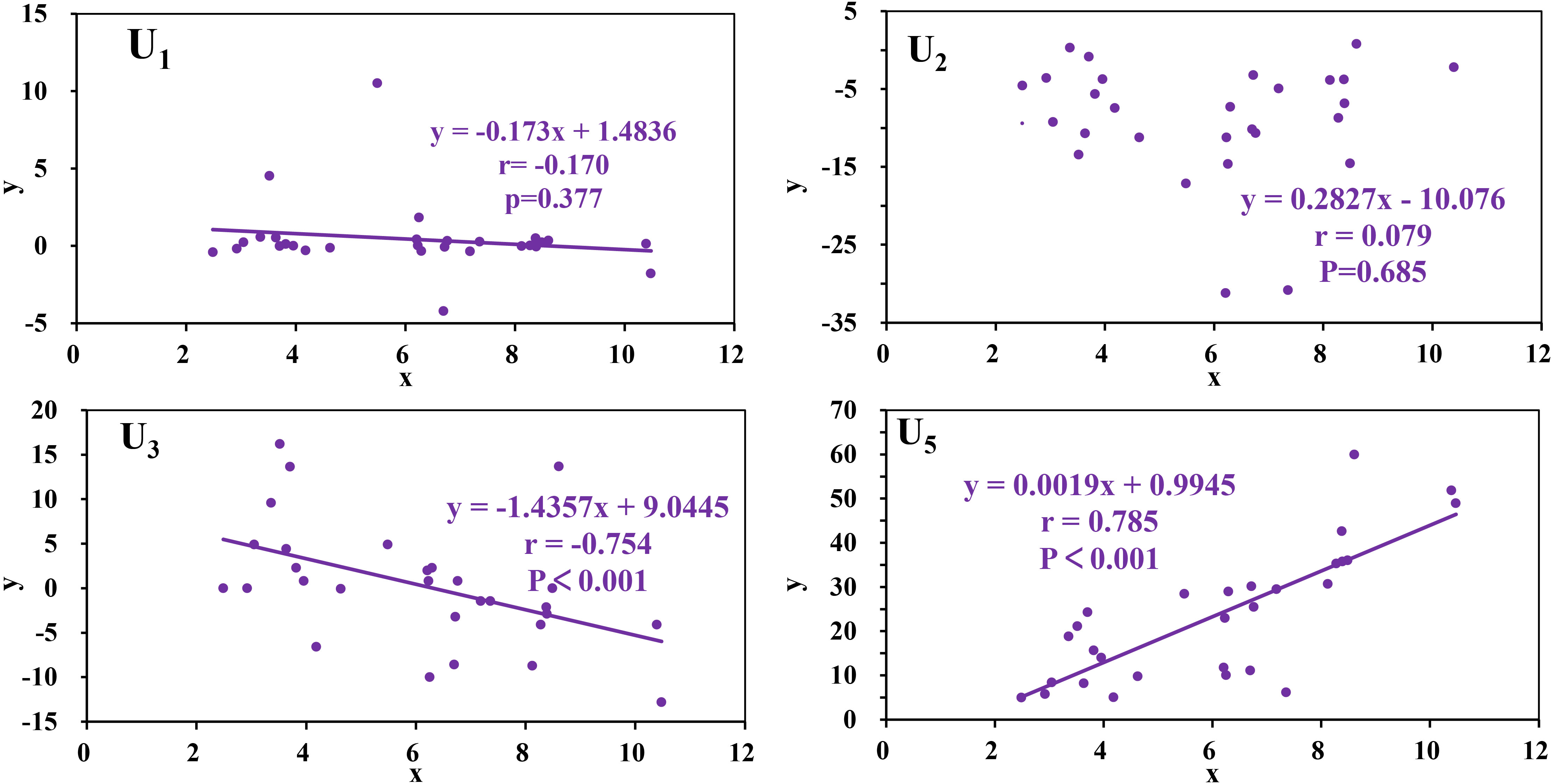
Figure 8 Correlation diagram of comprehensive evaluation vulnerability results and single factor index Ui linear regression (x in the figure is the comprehensive evaluation erosion vulnerability index, y is the original data of the factor index).
In practical research, coastal vulnerability is the degree of vulnerability based on the morphological characteristics of the coast itself, and the characteristics are affected by conditions such as coastal dynamics and human activities. Therefore, based on the state of coastal morphological factors, we conducted a partial correlation analysis between the vulnerability and the vulnerability of the remaining index factors. The results showed that the rate of change of the isobath (U2) was significantly negatively correlated with coastal vulnerability ( r =-0.58, p < 0.01), indicating that the greater the rate of change of the isobath was, the greater the degree of coastal vulnerability. The interannual downward rate of erosion of the beach (U3) was strongly negatively correlated with the degree of coastal vulnerability ( r =-0.75, p < 0.01); that is, the greater the interannual beach downward erosion rate was, the greater the vulnerability of the coast. The remaining indicators and the CVI were not obviously linearly related ( Figure 8 ). Therefore, the interannual downward erosion rate and isobath change rate of the beach were the main controlling factors in the vulnerability of coastal erosion, and the other factors were secondary factors.
5.1.1. Coastal morphological factors
Coastal morphological factors are important indicators reflecting the intrinsic characteristics of the coast. The width and slope of the beach can determine the reflection ability of waves, and the reflection ability of waves reflects the characteristics of erosion; that is, the erosion intensity of waves on the beach increasesWangYonghong ( Wang, 2018 ) and results in a higher erosion vulnerability. In contrast, a gentler slope and a wider beach result in lower vulnerability.
Figure 7B shows that the CVI curve was similar to the beach width U5 curve but had an opposite relationship with the slope; that is, a larger slope corresponds to a narrower beach width, which corresponds to a relatively lower vulnerability. In contrast, for flat and wider beaches, the slope was mostly gentle, and the vulnerability was relatively higher. There was a certain gap in the evaluation of individual factors because vulnerability was not presented according to the relationship of a single factor under the superimposed influence of the comprehensive factor indicators, which was consistent with the results of Wang (2018) in their assessment of erosion vulnerability along the Yangtze River Delta. Figure 9 shows that the proportion of coastal morphological factors in each segment was different to a certain extent; in general, coastal morphological factors played a secondary role in the vulnerability of each segment.
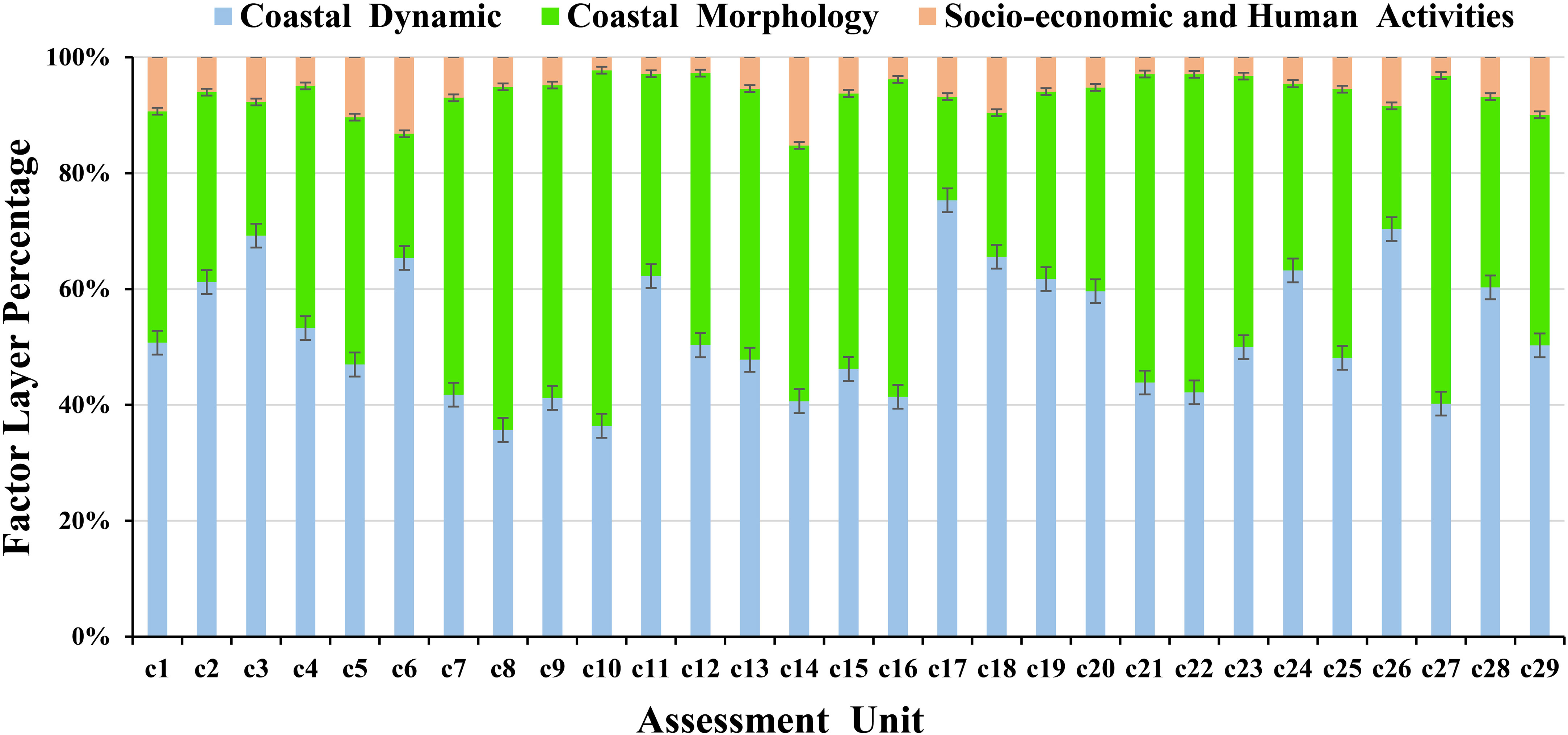
Figure 9 Map of the proportion of coastal morphology, coastal dynamics, and socioeconomic and human activity factors in each assessment unit.
5.1.2. Coastal dynamic factors
The coastal dynamic factors reflect the dynamic change characteristics of the external factors affecting the performance of the coast itself. In this assessment, the coastal vulnerability was characterized by erosion and siltation changes in the position of the supratidal beach, intertidal shoreline and subtidal nearshore sea floor and could better and more comprehensively reflect the vulnerability of the coast itself under the influence of the external environment. The assessment results showed that the coastal dynamic factors in the vulnerability of each segment were the main controlling effects overall ( Figure 9 ). Compared with coastal morphological factors and socioeconomic and human activity factors, they had a greater impact, which was consistent with the research by Hoque et al. (2019) , Sarwar (2013) , and Roy and Mahmood (2016) and most of the studies believe that coastal dynamic factors such as shoreline change rate are the key factors of coastal vulnerability.
Figure 7A shows that the interannual downward rate erosion of the beach (U3) was inversely proportional to the CVI curve; in addition, there was no obvious corresponding relationship under the influence of other comprehensive superposition. Our previous index weight evaluation showed that the coastal dynamic factor had a higher weight; in particular, the change rate of the shoreline (U1) had the highest weight value, but it did not have a dominant position in the comprehensive impact of vulnerability. This result was related to the evaluation under the condition of synthesizing various indicators and factors and reflected the comprehensive scientific nature of the CVI method.
5.1.3. Socioeconomic and human activity factors
The integration of socioeconomic and human activity factors enhances the reliability and scientificity of previous erosion vulnerability models that considered only coastal shape or coastal dynamic components ( Jana and Bhattacharya, 2013 ; Ahmed et al., 2018 ). The socioeconomic and human activity factors accounted for the smallest proportions in each evaluation unit and had the smallest impact value overall. The population density (U8) was generally inversely related to the CVI ( Figure 7C ), which indicated that in anthropogenic activities, the more developed tourism and frequent fishing activities are, the greater the vulnerability of the coast. However, the construction of coastal engineering, such as artificial islands, has had a greater impact on vulnerability than the straight section without engineering construction, which showed a state of moderate vulnerability; therefore, socioeconomic and human activity can increase the degree of coastal vulnerability.
5.2. Coastal types and the CVI
Different types of coastal areas have different erosion vulnerabilities, and even the same type of coastal area will show different results under the combined effect of different factors. There were large ranges of coral reefs (c2-c5) on the coast north of Tanmen Port in Qionghai. The multiyear coastline change rate and vulnerability curve are shown in Figure 10 . The c2-c4 section progressed with siltation overall, and the corresponding vulnerabilities were all below the low level, which had a certain relationship with the length of the reef flat in the coral reef disk, the roughness of the reef surface, and the reef crown on the wave propagation deformation. When the waves moved to the reef crown position, the wave energy was reduced due to its water blocking effect, causing the wave to increase water. When moving to the reef flat section, the water depth of the reef flat section was shallow, the existence of the large roughness of the reef surface reduced the wave water value, and the wave energy was further reduced. Therefore, the existence of coral reefs has a great energy dissipation effect on wave waterChaoShao ( Shao, 2016 ). It is a natural revetment barrier, reducing wave scour and erosion, and the coast was protected to a certain extent and showed low vulnerability.
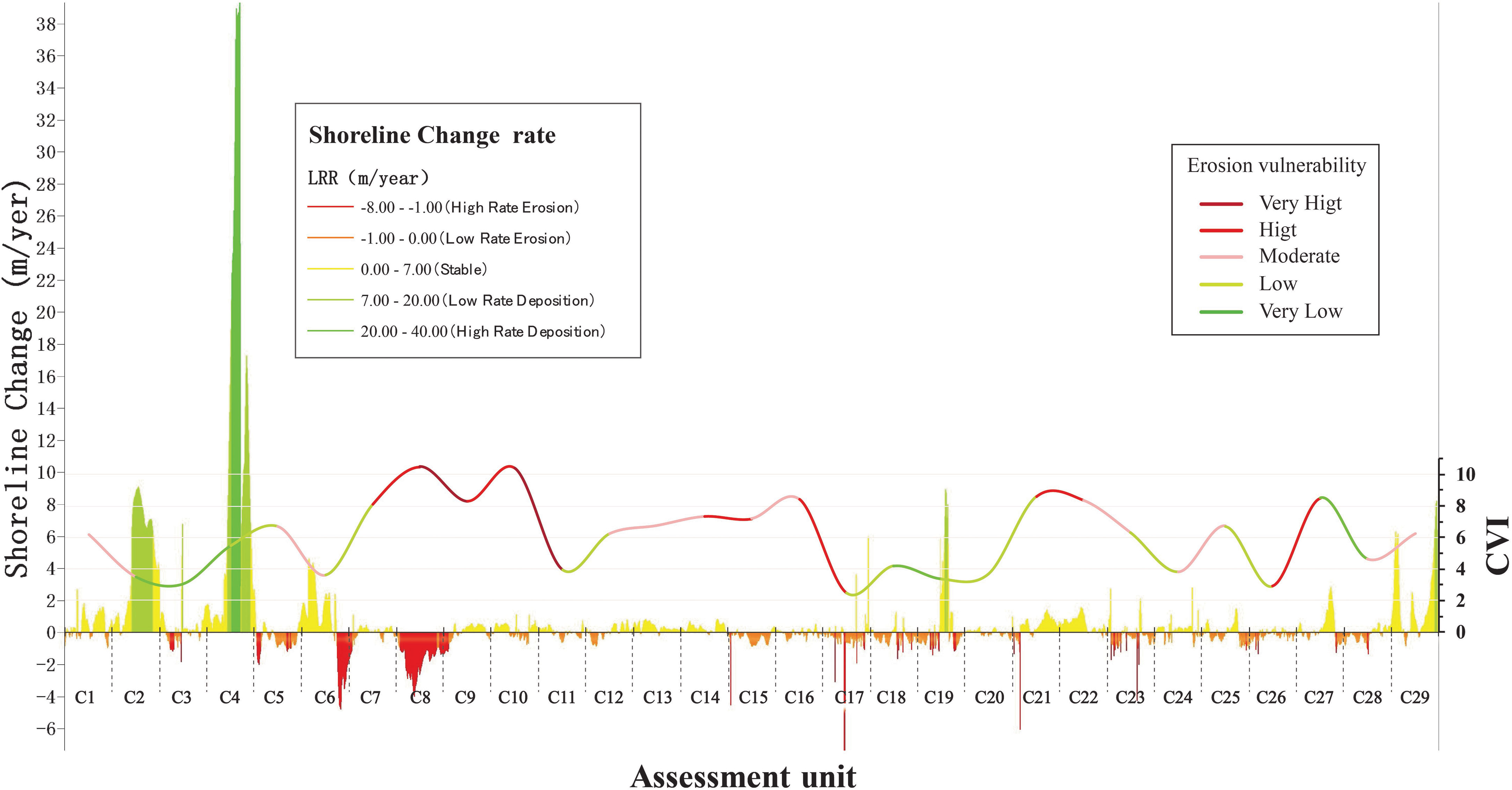
Figure 10 Shoreline change rate and vulnerability curve of different typical coasts (The left side is the legend of Change rate of shoreline, and the right side is the vulnerability index curve of the corresponding section).
The multiyear average rate of change in the c5 section was eroded, and the corresponding vulnerability was moderate vulnerability, which was related to the distribution of 2 artificial islands and the Tanmen port in the c5 coral reef section. Affected by the construction of artificial islands and the fishing activities in the port, the hydrodynamic environment in this area has become complicated, the coral reefs have been degraded, the natural barrier protection of the coral reefs has been reduced, erosion has occurred, and vulnerability has become moderate. In general, the presence of coral reefs has a protective effect on the coast, leaving the adjacent coast with relatively low vulnerability.
The estuarine segment (c8-c10) is the place with the highest degree of vulnerability in the study area, and distributed on both sides of the estuary, which is positively correlated with its erosion intensity. There is a two-way interaction between river runoff and tidal current at the estuary. The flow direction is opposite at high tide and same direction at low tide, forming a complex hydrodynamic environment, indicating that the hydrodynamic interaction at the estuary of the river under natural conditions is the main reason for the high vulnerability of the adjacent coast, which is consistent with the results of Roy et al (2021) study.
5.3. Vulnerability verification
In many vulnerability assessment studies, the reliability of the assessment results is verified by the area under the curve (AUC) under the receiver operating characteristic (ROC) curve, which has been widely used and verified by scholars ( Hoque et al., 2021b ; Rahman et al., 2019 ; Cao et al., 2022a ). By calculating the true positive rate (TPR) and the false positive rate (FPR) as the y axis to draw the characteristic curve, the size of the AUC can be calculated as the probability of verification.
where TP (true positives): the number of observed erosion locations accurately identified; FP (false positives): the number of erroneously identified erosion locations in non-eroded regions; TN (true negatives): correctly identified non-erosion locations number of; FN (false negatives): the number of misidentified non-eroded locations in eroded regions ( Roy et al., 2021 ).
We used the erosion points and non-erosion points determined by 390 observation points ( Figure 11A ) in the field survey as the validation dataset and the ROC curve in IBM SPSS Statistics 27 software to generate the prediction rate curve. The AUC of the prediction rate was 0.689, namely, the vulnerability assessment reached an accuracy of 68.9% ( Figure 11B ). The assessment results can provide important references for the development and management of the Hainan Qiongdong coastal zone and the ecological restoration of tropical coral reef biological coasts.
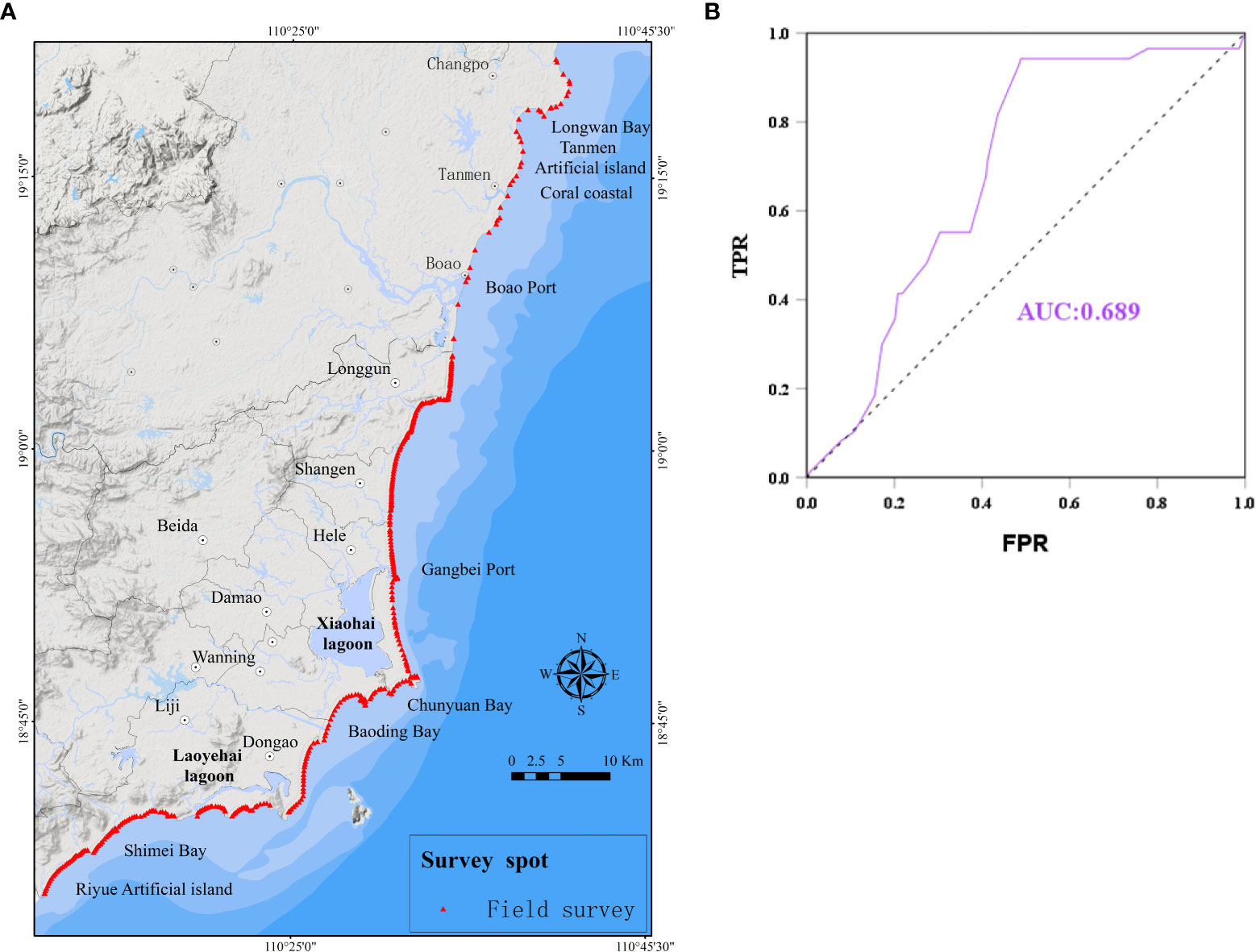
Figure 11 (A) Spatial distribution of field survey sites in 2021; (B) ROC curve and AUC = 0.689.
6. Conclusion
In this paper, 8 small-scale typical indicators, such as the change rate of the shoreline (U1) and the rate of change of the isobath (U2), were comprehensively selected, and the combination of DM and TEM was used to calculate the weight of comprehensive evaluation indicators, combined with GIS and the CVI to quantitatively evaluate the temporal and spatial distribution characteristics of coastal erosion vulnerability in Qionghai-Wanning, Qiongdong, Hainan.
(1) Most of the coastal erosion was in the state of moderate vulnerability or above, accounting for approximately 59% of the total shore section, of which the high-vulnerability section exceeded 31%. The coasts with higher vulnerability were mainly distributed in the Wanquan Estuary of Qionghai and some coasts of Cape Bay, and a few straight coasts also showed a state of higher vulnerability. The moderately vulnerable coasts were scattered, mainly in the inner bays of some headlands. The lower vulnerability areas accounted for approximately 41% of the Qionghai-Wanning coast, and they mainly distributed in coral reefs, headlands formed by bedrock, hard engineering and other locations.
(2) Under the influence of no control variables, the interannual downward rate erosion of the beach (U3) was negatively correlated with vulnerability, the beach width (U5) was highly positively correlated with vulnerability, and the rest showed an insignificant linear relationship with the CVI. Under the control variables, coastal vulnerability was based on the comprehensive influence of coastal morphological factors, showing that the rate of change of the isobath (U2) and the interannual downward erosion rate of the beach (U3) were highly negatively correlated with vulnerability. Therefore, the interannual downward erosion rate of the beach and the rate of change of the isobath were the main factors affecting the vulnerability to coastal erosion, and the other indicators were secondary factors. In addition, there were large coral reefs distributed north of Qionghai Tanmen as a natural barrier for revetment. The reason is that the length of the reef flat in the coral reef plate, the roughness of the reef surface and the reef crown can reduce the wave erosion and reduce the erosion of the coral reef coast, resulting in lower vulnerability, and the complex hydrodynamic environment, the estuarine coast presents a state of high vulnerability.
(3) The vulnerability was verified by the AUC value under the ROC curve, and the vulnerability assessment reached an accuracy of 68.9%. The assessment results provide a scientific basis for the restoration of coral reef biological coastal ecology and the development and management of the Qiongdong coastal zone.
Data availability statement
The original contributions presented in the study are included in the article/ Supplementary Material . Further inquiries can be directed to the corresponding authors.
Author contributions
Designed the study, wrote the main manuscript and prepared all figures: GF; contributed to the improvement of the manuscript: CC and KF; investigate: YS and KY; figure and software: GF, YS, and KY; all authors reviewed the manuscript. All authors contributed to the article and approved the submitted version.
This research was funded by the China Geological Survey Project: Qionghai-wanning Coastal Zone Comprehensive geological survey Project (Grant Nos. ZD20220608), the National Natural Science Foundation of China (Grant No. 42076058, 41930538), the Scientific Research Foundation of Third Institute of Oceanography, MNR (Grant No. 2019006).
Acknowledgments
The authors would like to express their sincere thanks to Huilong Xu, Fei Tan who have offered support.
Conflict of interest
The authors declare that the research was conducted in the absence of any commercial or financial relationships that could be construed as a potential conflict of interest.
Publisher’s note
All claims expressed in this article are solely those of the authors and do not necessarily represent those of their affiliated organizations, or those of the publisher, the editors and the reviewers. Any product that may be evaluated in this article, or claim that may be made by its manufacturer, is not guaranteed or endorsed by the publisher.
Supplementary material
The Supplementary Material for this article can be found online at: https://www.frontiersin.org/articles/10.3389/fmars.2022.1061769/full#supplementary-material
Ahmed A., Nawaz R., Drake F., Woulds C. (2018). Modelling land susceptibility to erosion in the coastal area of Bangladesh: A geospatial approach. Geomorphol. 320, 82–97. doi: 10.1016/j.geomorph.2018.08.004
CrossRef Full Text | Google Scholar
Alam A., Sammonds P., Ahmed B. (2020). Cyclone risk assessment of the cox’s bazar district and rohingya refugee camps in southeast Bangladesh. Sci. Total Environ. 704, 135360. doi: 10.1016/j.scitotenv.2019.135360
PubMed Abstract | CrossRef Full Text | Google Scholar
Alberico I., Amato V., Aucelli P., D’Argenio B., Di Paola G., Pappone G. (2012). Historical shoreline changes of the sele plain (southern italy): the 1870-2009 time window. J. Coast. Res. 28 (6), 1638–1647. doi: 10.2112/J.COASTRES-D-10-00197.1
Andrade T. S., Oliveira Sousa P. H. G., Siegle E. (2019). Vulnerability to beach erosion based on a coastal processes approach. Appl. Geogr. 102, 12–19. doi: 10.1016/j.apgeog.2018.11.003
Annelies B., das Neves L., de Nocker L., Ali D., Koen C.. (2021). A methodological framework of quantifying the cost of environmental degradation driven by coastal flooding and erosion: A case study in West Africa. J. Disaster Risk Reduction. 01 (54), 2212–4209. doi: 10.1016/j.ijdrr.2020.102022
Appeaning A. K. (2013). Shoreline morphological changes and the human factor, case study of Accra Ghana. J. Coast. Conserv. 17, 85–91. doi: 10.1007/s11852-012-0220-5
Compiled by China Meteorological Administration (2021). Tropical of cyclone yearbook Vol. 08 (China Meteorological Press), 15–193.
Google Scholar
Barragan J. M., Andreis M. (2015). Analysis and trends of the world’s coastal cities and agglomerations. Ocean Coast. Manage. 114, 11–20. doi: 10.1016/j.ocecoaman.2015.06.004
Bathi J. R., Das H. S. (2016). Vulnerability of coastal communities from storm surge and flood disasters. Int. J. Environ. Res. Publ. Health 13 (2), 239. doi: 10.3390/ijerph13020239
Boak E. H., Turner I. L. (2005). Shoreline definition and detection: A review. J. Coast. Res. 21 (4), 688–703. doi: 10.2112/03-0071.1
Boruff B. J., Emrich C., Cutter S. L. (2005). Erosion hazard vulnerability of US coastal counties. J. Coast. Res. 21 (5), 932–942. doi: 10.2112/04-0172.1
Bruun P. (1962). Sea-Level rise as a cause of shore erosion. J. Waterways Harbours Divisions. 88, 117–130. doi: 10.1061/JWHEAU.0000252
Cabrera J. S., Lee H. S. (2019). Flood-prone area assessment using GIS-based multi-criteria analysis: A case study in davao oriental, Philippines. Water. 11, 2203. doi: 10.3390/w11112203
Cabrera J. S., Lee H. S. (2020). Flood risk assessment for davao oriental in the Philippines using geographic information system-based multi-criteria analysis and the maximum entropy model. J. Flood Risk Management. 13, e12607. doi: 10.1111/jfr3.12607
Cai F., Cao C., Qi H. S., Lei G., Liu JH., Zhu K., et al. (2022). Rapid migration of Chinese mainland coastal erosion vulnerability due to anthropogenic changes. J. Environ. Management. 319, 115632. doi: 10.1016/j.jenvman.2022.115632 1–15
Cai F., Su X. Z., Cao C., Lei G., Liu JH., Qi HS., et al. (2019). China Coastal erosion vulnerability assessment and demonstration application. Beijing Ocean Press 08, 241–247.
Cai F., Su X. Z., Liu J. H., Li B., Lei G. (2008). Coastal erosion problems and preventive countermeasures in my country under the background of global climate change. Prog. Natural Sci. 10), 1093–1103.
Cao C., Cai F., Qi H., Liu JH., Lei G., Zhu K., et al. (2022a). Coastal erosion vulnerability in mainland China based on fuzzy evaluation of cloud models. Front. Mar. Sci. 8. doi: 10.3389/fmars.2021.790664
Cao C., Zhu K., Cai F., Qi H. S., Liu J. H., Lei G., et al. (2022b). Vulnerability evolution of coastal erosion in the pearl river estuary great bay area due to the influence of human activities in the past forty years. Front. Mar. Sci. 9, 847655. doi: 10.3389/fmars.2022.847655
Chen J. Y., Xia D. X., Yu Z. Y., Cai F. (2010). Summary of coastal erosion in China (Beijing: Beijing China Ocean Press), 320–324.
Chen J. Y., Shen H. T., Yun C. X. (1988). Dynamic process and geomorphological evolution of the Yangtze estuary (Shanghai: Shanghai Science and Technology Press), 158–189.
Chen S. L., Zhang G. A., Yang S. L., Yu Z. Y. (2004). Temporal and spatial variation of suspended sediment concentration and sediment resuspension in the waters of the Yangtze estuary. Chin. J. Geography. 02, 260–266. doi: 10.11821/xb200402012
Chu J. L., Gao S., Xu J. G. (2005). Risk and safety evaluation methodologies for coastal systems: A review. Mar. Sci. Bulletin. 24 (3), 80–87. doi: 10.1111/j.1745-7254.2005.00209
Deepika B., Avinash K., Jayappa K. S. (2013). Shoreline change rate estimation and its forecast: remote sensing, geographical information system and statistics-based approach. Int. J. Environ. Sci. Technol 11 (2) , 395–416. doi: 10.1007/s13762-013-0196-11-22
Dolan A. H., Walker I. J. (2006). Understanding vulnerability of coastal communities to climate change related risks. J. Coast. Res. 39, 1316–1323. doi: 10.2307/25742967
Dominguez L., Anfuso G., Gracia F. J. (2005). Vulnerability assessment of a retreating coast in SW Spain. Environ. Geology. 47 (8), 1037–1044. doi: 10.1007/s00254-005-1235-0
Dou X., Song J., Wang L., Tang B., Xu S., Kong F., et al. (2017). Flood risk assessment and mapping based on a modified multi-parameter flood hazard index model in the guanzhong urban area, China. Stoch. Environ. Res. Risk Assess. 32, 1131–1146. doi: 10.1007/s00477-017-1429-5
Gornitz V. (1991). Global coastal hazards from future sea level rise. Global Planetary Change. 89 (4), 379–398. doi: 10.1016/0921-8181(91)90118-G
Hagedoorn L. C., Appeaning A. K., Koetse M. J., Kinney K., van Beukering P. J. H.. (2021). Angry waves that eat the coast: An economic analysis of nature-based and engineering solutions to coastal erosion. Ocean Coast. Management. 11, 0964–5691. doi: 10.1016/j.ocecoaman.2021.105945
Hoque M. A. A., Pradhan B., Ahmed N., Ahmed B., Alamri A. M. (2021a). Cyclone vulnerability assessment of the western coast of Bangladesh, geomatics, nat. Hazards Risk. 12 (1), 198–221. doi: 10.1080/19475705.2020.1867652
Hoque M. A. A., Pradhan B., Ahmed N., Roy S. (2019). Tropical cyclone risk assessment using geospatial techniques for the eastern coastal region of Bangladesh. Sci. Total Environ. 692, 10–22. doi: 10.1016/j.scitotenv.2019.07.1320048-9697
Hoque M. A. A., Pradhna B., Ahmed N., Sohel M. S. I. (2021b). Agriculturla drought risk assessment of northern new south Wales, Australia using geospatial techniques. Sci. Total Environ. 756, 143600. doi: 10.1016/j.scitotenv.2020.143600
Huang H., Chen Z., Huang L. D., Wang X., Niu W. T., Tian J. J., et al. (2019). China Coral reef status report. Coral Reef Branch China Pacific Soc. , 32–37.
Jana A., Bhattacharya A. K. (2013). Assessment of coastal erosion vulnerability around midnapur-balasore coast, Eastern India using integrated remote sensing and GIS techniques. J. Indian Soc. Remote Sensing. 41 (3), 675–686. doi: 10.1007/s12524-012-0251-2
Ji R. Y., Luo X. L., Lu Y. J., Luo Z. R. (2007). Analysis of coastal erosion characteristics and main causes of hainan island. Proc. 13th China Ocean (Coastal) Eng. Symposium. 09, 374–377.
Jones A., Phillips M. (2011). Disappearing destinations: Climate change and the future challenges for coastal tourism. cabi, London. Publications review/Annals Tourism Res. 39, 2220–2226. doi: 10.1016/j.annals.2012.07.014
Kumar A. A., Kunte P. D. (2012). Coastal vulnerability assessment for chennai, east coast of India using geospatial techniques. Natural Hazards. 64 (1), 853–872. doi: 10.1007/s11069-012-0276-4
Liu X. X. (2015). Coastal evolution and intrusion vulnerability assessment of abandoned yellow river delta [Master’s thesis]. Shanghai East China Normal University 06, 2–4.
Liu H. W., Sun X. M., Wen D. G., Fang C., Hu Y. Z., Ma Z., et al. (2013). Vulnerability evaluation of the caofeidian coastal area based on coastal vulnerability index method. Hydrogeology Eng. Geology. 40 (3), 105–109. doi: 10.16030/j.cnki.issn.1000-3665.2013.03.013
Mangor K., Dronen N. K., Kaergaard K. H., Kristensen S. E. (2017). Shoreline management guidelines. Netherlands. DHI Water Environment. 294, 153–154.
Mattei G., Troisi S., Aucelli P. P., Pappone G., Peluso F., Stefanile M. (2018). Sensing the submerged landscape of nisida roman harbour in the gulf of naples from integrated measurements on a USV. Water. 10, 1686. doi: 10.3390/w10111686
Merlotto A., B´ertola G. R., Piccolo M. C. (2016). Hazard vulnerability and coastal erosion risk assessment in necochea municipality, buenos aires province. Argentina. J. Coast. Conserv. 20 (5), 351–362. doi: 10.1007/s11852-016-0447-7
Mohamed E-S. E-M., Ali S., Moursy F. E., Sharaky A., Saleh N.. (2021). Coastal erosion risk assessment and applied mitigation measures at ezbet elborg village, Egyptian delta. Ain Shams Eng. J 13 (3), 3–6. doi: 10.10.1016/j.asej.2021.10.016
Mosadeghi R., Warnken J., Tomlinson R., Mirfenderesk H.. (2019). Comparison of FuzzyAHP and AHP in a spatial multi-criteria decision making model for urban land-use planning. Comput. Environ. Urban Syst. 49, 54–65. doi: 10.1016/j.compenvurbsys.2014.10.001
Narra P., Coelho C., Sancho F., Escudero M., Silva R. (2019). Coastal hazard assessments for sandy coasts: Appraisal of five methodologies. J. Coast. Res. 35 (3), 574–589. doi: 10.2112/JCOASTRES-D-18-00083.1
Narra P., Coelho C., Sancho F., Palalane J. (2017). CERA: An open-source tool for coastal erosion risk assessment. Ocean Coast. Manage. 142, 1–14. doi: 10.1016/j.ocecoaman.2017.03.013
Pan Y., Yin S., Chen Y. P., Yang Y. B., Xu C. Y., Xu Z. S. (2022). An experimental study on the evolution of a submerged berm under the effects of regular waves in low-energy conditions. Coast. Eng. 176, 104169. doi: 10.1016/j.coastaleng.2022.104169
Parvin G. A., Takahashi F., Shaw R. (2008). Coastal hazards and community-coping methods in Bangladesh. J. Coast. Conserv. 12 (4), 181–193. doi: 10.1007/s11852-009-0044-0
Rahman M., Chen N. S., Islam M. M., Dewan A., Iqbal J., Muhammad R., et al. (2019). Flood susceptibility assessment in Bangladesh using machine learning and multi-criteria decision analysis. Earth Syst. Environment. 3, 585–601. doi: 10.1007/s41748-019-00123-y
Rodgers J. L., Nicewander W. A. (1988). Thirteen ways to look at the correlation coefficient. Am. Statis tician. 42 (1), 59–64. doi: 10.1080/00031305.1988.10475524
Roy S., Mahmood R. (2016). Monitoring shoreline dynamics using landsat and hydrological data: A case study of sandwip island of Bangladesh. Pennsylvania Geographer 54 (2), 20–41.
Roy S., Pandit S., Papia M., Rahman M. M., Ocampo J. C. O. R., Razi M. A., et al. (2021). Coastal erosion risk assessment in the dynamic estuary: The meghna estuary case of Bangladesh coast. Int. J. Disaster Risk Reduction. 07-14, 2212–4209. doi: 10.1016/j.ijdrr.2021.102364
Saaty T. L. (1980). The analytical hierarchy process (Great Britain: Mcgraw-Hill), 52–58.
Saffaria S., Mahmoudi A., Shafiee M., Jasemi M., Hashemi L. (2020). Measuring the effectiveness of AHP and fuzzy AHP models in environmental risk assessment of a gas power plant. Hum. Ecol. Risk Assess. 27 (5), 1227–1238. doi: 10.1080/10807039.2020.1816809
Sarwar M. G. M. (2013). “Sea-Level rise along the coast of Bangladesh,” in Disaster risk reduction approaches in Bangladesh . Eds. Shaw R., Mallick F., Islam A. (Tokyo: Springer), 217–231.
Shao C. (2016). Study on coastal erosion and adaptive management of beach-coral reef. [Master’s thesis]. Third Institute Oceanography State Oceanic Administration. 50–63. Available at: https://kns.cnki.net/KCMS/detail/detail.aspx?dbname=CMFD201701&filename=1016256149.nh
Shi P., Kasperson R. (2015). World atlas of natural disaster risk (Berlin: Springer-Verlag), 10–15.
Swami D., Parthasarathy D. (2021). Dynamics of exposure, sensitivity, adaptive capacity and agricultural vulnerability at district scale for maharashtra. India Ecol. Indic. 121, 107206. doi: 10.1016/j.ecolind.2020.107206
Thampanya U., Vermaat J. E., Sinsakul S., Panapitukkuld N.. (2006). Coastal erosion and mangrove progradation of southern Thailand. Estuarine Coast. Shelf Sci. 68 (1), 75–85. doi: 10.1016/j.ecss.2006.01.011
Thieler E. R., Hammar-Klose E. S. (2000). National assessment of coastal vulnerability to sea-level rise, preliminary results for the US Atlantic coast. Survey USGS. 28–35. doi: 10.9774/GLEAF.978-1-909493-38-4_2
Tian H. B., Yin P., Yang F. L. (2016). An analysis of erosional characteristics of the sandy coast in the eastern part of wanning, hainan. Mar. Geology Quaternary Geology. 38 (4), 44–55. doi: 10.16562/j.cnki.0256-1492.2018.04.004
Wang Y. H.. (2018). Coastal dynamic geomorphology. Beijing Sci. Press 05, 99–103.
Wang X. T., Zhang W. G., Yin J., Wang J., Ge J. Z., Wu J. P., et al. (2021). Assessment of coastal erosion vulnerability and socio-economic impact along the Yangtze river delta. Ocean Coast. Management. 201, 164–191. doi: 10.1016/j.ocecoaman.2021.105953
Wijitkosum S., Sriburi T. (2019). Fuzzy AHP integrated with GIS analyses for drought risk assessment: A case study from upper phetchaburi river basin, Thailand. Water. 11 (5), 939. doi: 10.3390/w11050939
Xia D. X., Wang W. H., Wu G. Q., Cui J. Q., Li F. L., et al. (1993). Coastal erosion in China. Acta Geographica Sinica. 48 (5), 468–475. doi: 10.11821/xb199305010
Yang Z. X. (2013). Monitoring coastline changes and tidal flat reclamation in jiangsu using remote sensing technology. Yellow River. 1), 85–87. doi: 10.3969/j.issn.1000-1379.2013.01.027
Yoo G., Kim A. R., Hadi S. (2014). A methodology to assess environmental vulnerability in a coastal city: Application to Jakarta, Indonesia. Ocean Coast. Management. 102, 169–177. doi: 10.1016/j.ocecoaman.2014.09.018
Zou Z. H., Yun Y., Sun J. N. (2006). Entropy method for determination of weight of evaluating indicators in fuzzy synthetic evaluation for water quality assessment. J. Environ. Sci. 18 (5), 1020–1023. doi: 10.1016/S1001-0742(06)60032-6
Keywords: Hainan Island, coral reef coast, human activities, index method, coastal erosion vulnerability
Citation: Fu G, Cao C, Fu K, Song Y, Yuan K, Wan X, Zhu Z, Wang Z and Huang Z (2022) Characteristics and evaluation of coastal erosion vulnerability of typical coast on Hainan Island. Front. Mar. Sci. 9:1061769. doi: 10.3389/fmars.2022.1061769
Received: 05 October 2022; Accepted: 15 November 2022; Published: 20 December 2022.
Reviewed by:
Copyright © 2022 Fu, Cao, Fu, Song, Yuan, Wan, Zhu, Wang and Huang. This is an open-access article distributed under the terms of the Creative Commons Attribution License (CC BY) . The use, distribution or reproduction in other forums is permitted, provided the original author(s) and the copyright owner(s) are credited and that the original publication in this journal is cited, in accordance with accepted academic practice. No use, distribution or reproduction is permitted which does not comply with these terms.
*Correspondence: Chao Cao, [email protected] ; KaiZhe Fu, [email protected]
This article is part of the Research Topic
Hydrodynamics and Water Environment Characteristics in Coastal Areas under the Influences of Climate Change and Human Activities

Coastal Erosion
Wave action and flooding in coastal areas can wear down or carry away rocks, soils, and/or sands along the coast resulting in coastal erosion. Coastal storms that include large waves, storm surge, potentially in combination with high tides, create more damaging conditions to the coastline and any built structures that exist on the coastline. Seasonal changes occur due to late summer/fall hurricanes and winter storms. Coastal storm events often devastate barrier islands, which buffer the main coastlines from the storm energy. Rising sea levels and increasing intensity and frequency of coastal storms are making coastal erosion worse.
The videos explain how coastal erosion occurs and highlights the many ways damage is occurring to human infrastructure.
Drone video of extreme coastal erosion along Pacific Beach near San Francisco, CA.
Extreme coastal erosion along the shorelines of Lake Michigan in northern Indiana.
Great perspective on the problems and potential solutions along the coastline of South Carolina. (Watch the first few minutes for an introduction.)
Exercise 3 – Coastal Erosion
Use the resources below to answer the following questions:
- Coastal Erosion | U.S. Climate Resilience Toolkit
- Definitions of coastal terms – Coastal Wiki
- Watch the videos
- Coastal erosion is the wearing away and transportation of material away from one section of a coastline. T/F
- Coastal erosion removes material from the shoreline and redistributes it to other parts of the coastline. T/F
- Coastal erosion can also occur gradually over a long time due to sediment deficits. T/F
- Coastal erosion is reversible. T/F
- What is the main cause of erosion along a coastline?
- This feature along the coastline offers the best protection to the mainland from coastal storms.
- How do tropical storms impact coastal erosion?
- What is the estimated value of property loss each year due to coastal erosion in the US?
- These two coastlines of the US are experiencing the highest coastal recession.
Coastal Engineering
Coastline erosion around the world, particularly in large cities, is often at considerable risk due to coastal erosion. The erosion is often mitigated using a combination of coastal structures that can arrest the loss of sediment, redirect wave energy, or redirect the flow of sediment along coastlines.
Exercise 4 – Coastal Engineering
- Coastal Protection and Mitigation | Coastal Processes, Hazards, and Society (psu.edu)
- beachnourishment.wcu.edu
- What are the two types of strategies used to reduce or stop erosion along coastlines?
- These erosion mitigation strategies are cost-effective in the long run. T/F
- These erosion mitigation strategies help reduce coastal erosion permanently. T/F
- Identify the different methods below that represent hard mitigation strategies.
- Identify the different methods below that represent soft mitigation strategies.
Coastal Protection Using Hard Structures (psu.edu) shows different types of hard structures. Use Google Earth to navigate to the geographical locations shown below (Latitude (N) and Longitude (W)) and identify the different coastal erosion and protection structures in the area.
- 32.7692852 -79.9288478
- 32.7304813 -79.8359377
- 32.6787736 -79.8941089
- 32.686272 -79.8863391
- 25.7629516 -80.1274675
- 25.8053492 -80.1225144
Now navigate to Folly Beach County park at this link on Google Earth , and answer the following questions:
- What type of coastal structure is installed here?
- Which side of this structure is accumulating sediment?
- Which side of this structure is losing sediment?
- Based on the gain and loss of sediment next to the structure, what is the direction of longshore drift?
Using beach nourishment data for South Carolina, answer the following questions:
- Move your mouse cursor on the map and identify which coast of the US has the highest beach nourishment episodes.
- Move your mouse cursor on the map and identify which US state has the highest beach nourishment episodes.
- How many different episodes of beach nourishment have occurred in SC?
- What is the approximate cost (in 2020 cost) of all these beach nourishments (round off to the nearest million)?
- What is the approximate cost (in 2020 cost) of Folly Beach nourishments (round off to the nearest million)?
- What is the primary funding source for these beach nourishment projects?
- Explain if this source of funding may be reasonable. Give one each of Pro and Con rationale.
- Consult the US Climate Resilience Kit and list two non-structural solutions to stabilizing coastlines such as Folly Beach.
Environmental Geology Laboratory Copyright © 2021 by Dr. Vijay M. Vulava and colleagues at the Department of Geology and Environmental Geosciences, College of Charleston is licensed under a Creative Commons Attribution-NonCommercial-ShareAlike 4.0 International License , except where otherwise noted.
Share This Book
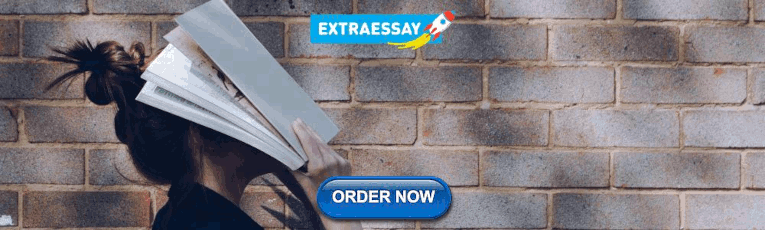
IMAGES
VIDEO
COMMENTS
Sigrid Martinez 02/11/21 Mr. Gonzales 4.2.2 Lab: Coastal Erosion Case Study After looking at the evidence and doing my own research I have reached the best conclusion for the civilians of "El C." As you take a look at the sketch below you will see a barrier island (like the village) that can be affected by sea-level fluctuations. On a short time scale, they are the result of interactions ...
View 4.2.2 Coastal Erosion Case Study Report.pdf from SCIENCES 165 at Stratford High School. Gallerano 1 Mallory Gallerano Coach Hall Earth, Wind, and Fire 2 February 2022 Coastal Erosion Case Study: ... lab 8 landforms.docx. Georgia State University. GEOG 1113. Erosion. Cliff. River delta. Landform. stumps. lab 8 landforms.docx.
presence of numerous coastal structures. The study area extends for about 1.5 km, inside there are numerous coastal structures, two river mouths and numerous houses built close to the shoreline. Keywords: coastal erosion, coastal erosion risk assessment, hazard, vulnerability, index-based methodology, coastal structures. 1 INTRODUCTION
View 4.2.2 Lab UT CECaseStudyPt3.docx from SCIENCE 123 at Taft High School. Introduction to Geoscience: Earth, Wind, and Fire NAME: _ The Village of El C. A Case Study in Coastal Erosion Part 3: Case
Shorelines are dynamic aspects of coastal ecosystems that alter in response to wave energy, storm events, and changes in sea level and sediment supply [].Shoreline changes are primarily caused by natural processes such as erosion and accretion, which can occur as a result of a range of short- and long-term events [].Storms, waves, tides, and winds are all short-term occurrences, whereas ...
Physical Processes in Estuaries pp 427-445 Cite as. Laboratory Studies on Cohesive Sediment Deposition and Erosion. A. J. Mehta 3 ; Conference paper; 508 Accesses. 47 Citations.
PDF | On Nov 30, 2013, Durusoju Hari Prasad and others published Coastal Erosion Studies—A Review | Find, read and cite all the research you need on ResearchGate
Purpose At the intersection of land and sea, coastal zones are unstable areas that can suffer intensive coastal erosion or sedimentation. The Yellow River Delta, which has experienced rapid land growth, is of great significance in soil fertility research. Materials and methods To qualitatively and quantitatively analyse the basic soil fertility characteristics of coastal zones, we sampled six ...
Many research studies have focused on developing the technique to be ready for real-world applications, and some studies have begun to investigate the performance of microbial application at coastal bluffs and sand dunes to mitigate coastal erosion (Phillips et al 2013, Imran et al 2019, Shahin et al 2020). Detailed information of the two case ...
Coastal erosion risk assessment. The study area was divided into 61 segments of 500 m length. For each segment the Coastal Erosion Risk Index was calculated. This index is based on the mathematical combination of two sub-indices within a GIS environment according the methodology proposed by Rangel-Buitrago and Anfuso (2015). These two sub ...
Charisma Rodriguez March 7, 2022 4.2.2 Lab: Coastal Erosion Lab Study Part 1: 1. The loss of displacement of land due to currents, waves, tides, and more. All coastlines are eroding 2. When the sea loses energy, it drops everything it's been carrying 3. With final report 4. Currents made by the waves 5. Main sources of sediment are coastal landforms, sinks are coastal dunes and submarine ...
Coastal Erosion Case Study. In the U., coastal erosion is responsible for roughly $500 million per year in coastal property loss, including damage to structures and loss of land. To mitigate coastal erosion, the federal government spends an average of $150 million every year on beach nourishment and other shoreline erosion control measures.
By Susan Enright.. The results of a collaborative research project led by a graduate student at the University of Hawai'i at Hilo have been published as a case study in the U.S. Climate Resilience Toolkit, a website where the public can find information and tools to understand and address climate risks.The website offers information from all across the United States federal government in one ...
Coastal erosion is a common phenomenon along the world's coasts. Studying it is complex because such studies must cover large portions of land, and it is necessary to understand the multiple processes that interact in each area, so it is important to recognize regional patterns that allow for defining representativeness in relation to the surrounding dynamics. Spatial statistics can be used ...
You can see the cement truck and boulders beyond the dark grey area if you expand the image. This page titled 4.2.2: Erosion below the Auxiliary Spillway is shared under a not declared license and was authored, remixed, and/or curated by Dawn Sumner. Images of erosion, showing very rapid erosion at one nick point in particular.
View 4.2.2 Lab.docx from GEOSCIENCE 325C at University of Texas. 1 Madison Phillips Berdugo Geo 07 February 2024 4.2.2 Lab: Coastal Erosion Case Study After learning about "El C", I decided to do. AI Homework Help. Expert Help. Study Resources. Log in Join. 4.2.2 Lab.docx - 1 Madison Phillips Berdugo Geo 07 February... Doc Preview.
Introduction: In this lesson plan, students study methods for protecting coastlines. Coastal erosion is a serious problem, since almost half the U.S. population lives near or along the coast. Hurricanes and other strong storms cause a lot of damage to a beach in a short time. In this lesson, students learn that.
In the 1970s and 1980s, the coastal erosion of Hainan Island already affected approximately 53% of the coastline (Xia et al., 1993), and in the 1990s, the eroded coast of Hainan increased to 71.9%, and there were 64 eroded coasts on the whole island, and erosion was strong.There were 7 shore sections, of which 3 were located in the strongly eroded section of the study area, accounting for ...
Coastal Erosion Wave action and flooding in coastal areas can wear down or carry away rocks, soils, and/or sands along the coast resulting in coastal erosion. Coastal storms that include large waves, storm surge, potentially in combination with high tides, create more damaging conditions to the coastline and any built structures that exist on ...
The development of ecological restoration projects is unsatisfactory, and soil erosion is still a problem in ecologically restored areas. Traditional soil erosion studies are mostly based on satellite remote sensing data and traditional soil erosion models, which cannot accurately characterize the soil erosion conditions in ecological restoration areas (mainly plantation forests). This paper ...
View 4.2.2 Lab UT CECaseStudyPt3.pdf from ENGINERING 3142 at University of Texas, Arlington. Introduction to Geoscience: Earth, Wind, and Fire NAME: _ The Village of El C. ... A Case Study in Coastal Erosion Part 3: Case Study Report ... _____ Rubric for Coastal Erosion Lab 8 6 4 Clarity of Stated Recommendation Recommendation is clearly stated ...
Before you draw final conclusions about the cause of the April 24, 2015 event, view the following graph (4.2.3) that shows a combination of data collected at Axial Seamount since 1998 from numerous studies. Note that the 2015 event you've studied is included in the graph along with similar changes to the seafloor at two other times since 1998.
Lab Rubric-1.docx from GEOLOGY 302D at University of Texas. Rubric for 4.2.2. Coastal Erosion Lab 8 6 4 Recommendation is muddled, containing disjointed pieces of ideas. 2 Clarity of. ... Upload your study docs or become a member. View full document. Other related materials See more. ... University of Texas, Arlington. MAS 3319.